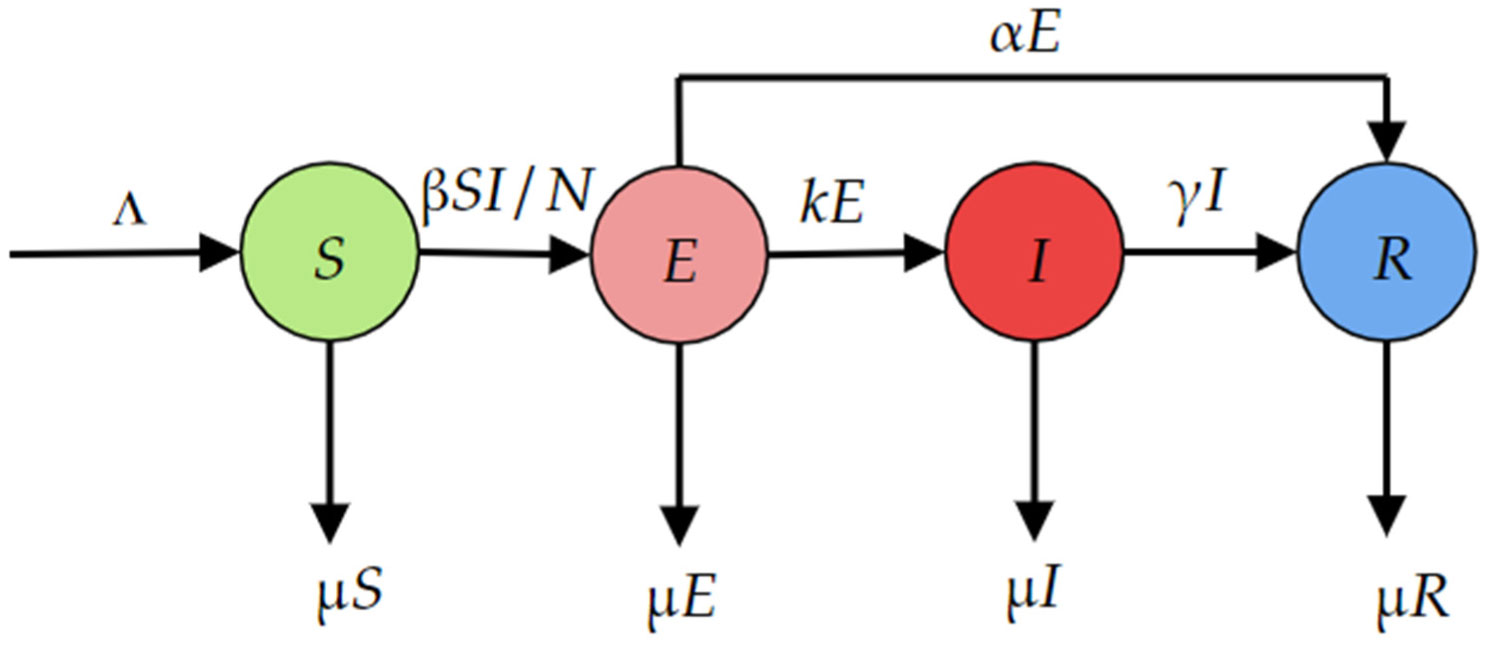
Tire pyrolytic char has the potential to be used as a raw material/product in different applications. The only problem associated with the tire pyrolytic char is the presence of some impurities, which limits its potential for use as carbon black and as a precursor to producing activated carbon. Tire pyrolytic char contains high concentrations of Zn2+ and S, which are used as ingredients while manufacturing tires. Our main aim of this study was to perform leaching studies to remove of Zn2+ and SO42- from the tire pyrolytic char using a solution of H2O2 as a solvent and to perform kinetic studies further using the shrinking core model to determine the rate-limiting step for the leaching of Zn2+ and SO42-. Process variables such as stirring speed (rpm), solvent concentration (ppm), temperature (℃), and solid-to-liquid ratio (g/100 ml) were investigated. It was found that the leaching rate of Zn2+ and SO42- from the pyrolytic tire char increased with an increase in all the process variables except for the solid to liquid ratio, which had an opposite effect on the leaching rate. Fourier Transform Infrared Spectra (FTIR) analysis, SEM-EDS, and Xray diffraction (XRD) analysis were used to characterize the pyrolytic tire char before and after the leaching experiments. FTIR showed that sulfur-containing functional groups disappeared after leaching with a solution of H2O2. SEM-EDS also showed a reduction in both Zn and S presence from the pyrolytic tire char after the leaching. XRD results showed that the raw tire pyrolytic char contained diffraction peaks associated with ZnO and ZnS, which disappeared after exposure to leaching. The kinetic parameters obtained showed that the rate-limiting step for the leaching of Zn2+ and SO42- from tire pyrolytic char was diffusion through the product layer. The regression coefficients obtained for diffusion through the product layer for both Zn2+ and SO42- were closer to 1 than values obtained for the chemical reaction between the fluid reactant with the solid at the surface of the solid particle. This was further confirmed by the apparent activation energies obtained for the leaching of Zn2+ and SO42- -11 kJ.mol-1 and 36 kJ.mol-1, respectively. Semi-empirical models for the leaching of Zn2+ and SO42- were developed to describe the leaching process.
Citation: Lucky Malise, Hilary Rutto, Tumisang Seodigeng, Linda Sibali. Leaching kinetics of ZN2+ and SO42- from tire pyrolytic char (TPC) using the shrinking core model[J]. Clean Technologies and Recycling, 2025, 5(1): 22-43. doi: 10.3934/ctr.2025002
[1] | Kenneth H. Karlsen, Süleyman Ulusoy . On a hyperbolic Keller-Segel system with degenerate nonlinear fractional diffusion. Networks and Heterogeneous Media, 2016, 11(1): 181-201. doi: 10.3934/nhm.2016.11.181 |
[2] | José Antonio Carrillo, Yingping Peng, Aneta Wróblewska-Kamińska . Relative entropy method for the relaxation limit of hydrodynamic models. Networks and Heterogeneous Media, 2020, 15(3): 369-387. doi: 10.3934/nhm.2020023 |
[3] | Piotr Biler, Grzegorz Karch, Jacek Zienkiewicz . Morrey spaces norms and criteria for blowup in chemotaxis models. Networks and Heterogeneous Media, 2016, 11(2): 239-250. doi: 10.3934/nhm.2016.11.239 |
[4] | Panpan Xu, Yongbin Ge, Lin Zhang . High-order finite difference approximation of the Keller-Segel model with additional self- and cross-diffusion terms and a logistic source. Networks and Heterogeneous Media, 2023, 18(4): 1471-1492. doi: 10.3934/nhm.2023065 |
[5] | Steinar Evje, Kenneth H. Karlsen . Hyperbolic-elliptic models for well-reservoir flow. Networks and Heterogeneous Media, 2006, 1(4): 639-673. doi: 10.3934/nhm.2006.1.639 |
[6] | Raul Borsche, Axel Klar, T. N. Ha Pham . Nonlinear flux-limited models for chemotaxis on networks. Networks and Heterogeneous Media, 2017, 12(3): 381-401. doi: 10.3934/nhm.2017017 |
[7] | Avner Friedman . PDE problems arising in mathematical biology. Networks and Heterogeneous Media, 2012, 7(4): 691-703. doi: 10.3934/nhm.2012.7.691 |
[8] | Hirotada Honda . Global-in-time solution and stability of Kuramoto-Sakaguchi equation under non-local Coupling. Networks and Heterogeneous Media, 2017, 12(1): 25-57. doi: 10.3934/nhm.2017002 |
[9] | Caihong Gu, Yanbin Tang . Global solution to the Cauchy problem of fractional drift diffusion system with power-law nonlinearity. Networks and Heterogeneous Media, 2023, 18(1): 109-139. doi: 10.3934/nhm.2023005 |
[10] | Stefan Berres, Ricardo Ruiz-Baier, Hartmut Schwandt, Elmer M. Tory . An adaptive finite-volume method for a model of two-phase pedestrian flow. Networks and Heterogeneous Media, 2011, 6(3): 401-423. doi: 10.3934/nhm.2011.6.401 |
Tire pyrolytic char has the potential to be used as a raw material/product in different applications. The only problem associated with the tire pyrolytic char is the presence of some impurities, which limits its potential for use as carbon black and as a precursor to producing activated carbon. Tire pyrolytic char contains high concentrations of Zn2+ and S, which are used as ingredients while manufacturing tires. Our main aim of this study was to perform leaching studies to remove of Zn2+ and SO42- from the tire pyrolytic char using a solution of H2O2 as a solvent and to perform kinetic studies further using the shrinking core model to determine the rate-limiting step for the leaching of Zn2+ and SO42-. Process variables such as stirring speed (rpm), solvent concentration (ppm), temperature (℃), and solid-to-liquid ratio (g/100 ml) were investigated. It was found that the leaching rate of Zn2+ and SO42- from the pyrolytic tire char increased with an increase in all the process variables except for the solid to liquid ratio, which had an opposite effect on the leaching rate. Fourier Transform Infrared Spectra (FTIR) analysis, SEM-EDS, and Xray diffraction (XRD) analysis were used to characterize the pyrolytic tire char before and after the leaching experiments. FTIR showed that sulfur-containing functional groups disappeared after leaching with a solution of H2O2. SEM-EDS also showed a reduction in both Zn and S presence from the pyrolytic tire char after the leaching. XRD results showed that the raw tire pyrolytic char contained diffraction peaks associated with ZnO and ZnS, which disappeared after exposure to leaching. The kinetic parameters obtained showed that the rate-limiting step for the leaching of Zn2+ and SO42- from tire pyrolytic char was diffusion through the product layer. The regression coefficients obtained for diffusion through the product layer for both Zn2+ and SO42- were closer to 1 than values obtained for the chemical reaction between the fluid reactant with the solid at the surface of the solid particle. This was further confirmed by the apparent activation energies obtained for the leaching of Zn2+ and SO42- -11 kJ.mol-1 and 36 kJ.mol-1, respectively. Semi-empirical models for the leaching of Zn2+ and SO42- were developed to describe the leaching process.
Coronavirus disease 2019 (COVID-19) is a contagious disease caused by severe acute respiratory syndrome coronavirus 2 (SARS-CoV-2). The virus was first identified in Wuhan, China, in December 2019, and it quickly spread to other parts of mainland China and many other countries [1]–[6]. Due to the rapid spread of the virus with consequences worldwide, the World Health Organization (WHO) declared a public health emergency of international concern on January 30, 2020, and a pandemic on March 11, 2020 [7],[8]. The transmission of COVID-19 has become a global health threat. As of April 17, 2020, there was a total of 2,230,439 COVID-19 cases reported worldwide, with 150,810 deaths and 564,210 recovered cases [9]. According to the WHO's COVID-19 global report, over 21.2 million people had been infected with the virus as of August 16, 2020, with over 761,779 deaths [10]. Moreover, till October 14, 2021, more than 239,426,728 cases of infection and at least 4,878,373 deaths have been confirmed in Africa, Asia, Europe, North America, Oceania, South America and Antarctica. This has become one of the deadliest pandemics in history according to the Center for Systems Science and Engineering (CSSE) at Johns Hopkins University (JHU) [11].
Studying and analyzing complex infectious disease models are difficult tasks and require some computational tools and mathematical approaches. In systems biology, mathematical presentations and computational simulations are effective tools and will help further understanding and future predictions about model dynamics. The models of COVID-19 are complicated, and they are required some mathematical tools to improve interventions and healthcare strategies. Recently, several studies about COVID-19 were suggested based on mathematical tools and computational simulations to understand this pandemic and its control approaches. In [12]–[14], the SEIR model was modified by several researchers to understand the impacts of social distancing, rapid testing, vaccination and contaminated objects in controlling the spread of COVID-19. One of the most important key elements in the spreading of COVID-19 is the basic reproduction number (also called basic reproductive ratio). This quantity, denoted by R0, represents the number of secondary infections induced by a single primary infection in a population where everyone is susceptible [15]. According to developed techniques of mathematical epidemiology models, R0 plays an important role for understanding epidemiological ideas and identifying key critical parameters for such models, and R0 may have different values for reported cases of coronavirus. According to a published study, the basic reproduction number was computed using incidence data in the city of Jakarta from March 3, 2020, to May 10, 2020. The median value of R0 was about 1.75 during the first interval. After the rigorous social distancing implemented, this value was about 1.22. In addition, the basic reproduction number has been calculated for different infectious disease models. Consequently, this quantity was calculated for a mathematical model of the CD8+ T-cell response to human T cell leukemia/lymphoma virus type I (HTLV-I) infection, given in [16].
According to published studies given in [14],[17],[18], the concept of sensitivity analysis has been presented to detect model sensitive parameters, including local sensitivities for each model state with respect to model parameters. Such sensitivities are computed by using three different methods: non-normalization, half-normalization and full-normalization. Accordingly, there was another method of sensitivity analysis given in [19]. They suggested the partial rank correlation coefficient (PRCC) technique to determine how the glioma-immune model output is affected by changes in specific parameter values.
Optimal control analysis is another effective method to identify the model critical parameters and to suggest control strategies. This technique has been used with the COVID-19 pandemic for deriving control policies. Recently, this technique has been used to find some control strategies for the COVID-19 pandemic in Indonesia, given in [20]. Then, there was a study about mathematical modeling with optimal control analysis for the spread of the COVID-19 pandemic with a case study of Brazil and cost-effectiveness; this was given in [21]. More recently, there was another approach that suggested a mathematical model with optimal control to highlight the negative impact of quarantine on diabetic people, with cost-effectiveness, presented in [22]. The reader can also find more relevant works about different approaches and mathematical models of the COVID-19 pandemic and its control strategies in [23]–[29].
Although there are many mathematical models that have been suggested for prediction of the COVID-19 pandemic, such models can be further improved and analyzed in terms of real data. Calculating the basic reproduction number with local sensitivities can improve the model outcomes further. An issue that has not been considered enough is elasticity of basic reproduction number with respect to model parameters. This helps us to identify the most critical model parameters in spreading this disease. Another issue of modeling such phenomena is validating the model results. Therefore, comparing the model predictions with real data can also indicate the accuracy and reliability of such models.
Estimating the model initial parameters for the suggested SEIR model is an important step forward in this study compared to the other existing studies about the confirmed cases of COVID-19 in the Kurdistan Region of Iraq. Therefore, we suggest the Metropolis–Hastings (MH) algorithm to estimate such model parameters, and then we use them in computational simulations. Such estimated parameters can also provide a good agreement between the model results and the daily infected cases. Another novelty here is the data collection of COVID-19 infected cases in the area that provides the future predictions and interventions. Accordingly, we have collected the daily confirmed cases of COVID-19 from January 10, 2021, to March 22, 2021. The collected real data here give us a great understanding about this disease in the area and suggested more interventions and strategies to control this pandemic. Interestingly, identifying the critical model parameters based on sensitivity and elasticity methods becomes another novelty for the suggested SEIR model.
This paper has some contributions. The main contribution is suggesting the simplified SEIR model for COVID-19 disease to predict the spreading of the disease in the Kurdistan Region of Iraq. We use this model to compare the model results and the daily infected cases more easily and correctly. Another contribution here is identifying the model critical parameters. Consequently, we suggest the local sensitivity methods to calculate the model sensitivities between the model variables and parameters. Additionally, the elasticity between the basic reproduction number, R0, and model parameters are also calculated and helps to identify the key critical parameters in spreading this disease.
The organization of this paper is as follows. In Section 2, we construct the SEIR model based on our assumptions and model transmissions. Using the next generation matrix, we derive the formula of the basic reproduction number, calculate the elasticity between R0 and model parameters and show the model local sensitivities based on the estimated values, as discussed in Section 3. We perform our computational model simulations of daily infected cases in Section 4. Finally, some conclusions and suggestions are given in Section 5.
Many infectious disease models have been suggested to understand the transmission rates of COVID-19. The SEIR model has been commonly applied in many infectious diseases. This model consists of four compartments, namely, susceptible S(t), exposed E(t), infected I(t) and recovered R(t) individuals. Several authors modified the SEIR model for studying the transmission of pathogens. Among them, in [30], a modified SEIR model was used to describe the 2009 influenza A (H1N1) pandemic within local regions in Japan. It was also used for the Ebola epidemic occurring in the West African nations of Guinea, Liberia and Sierra Leone [31]. In this paper, we use the SEIR model for COVID-19 disease that describes the spreading of COVID-19 in the Kurdistan Region of Iraq. Accordingly, we develop the previously published work given in [32], and we add a parameter that shows a rate of recovery of the individuals from the exposed class. The model network of SEIR shown in Figure 1 includes various compartments with their transmissions. The model compartments and transmission rates are defined in Table 1.
The chemical reactions of the model can be expressed as follows:
Furthermore, all parameters and initial states with their biological meanings are shown in Table 1. We use the concepts of standard chemical kinetics and the mass action law to derive a system of non–linear ordinary differential equations [33],[34]. Then, the system of nonlinear ordinary differential equations takes the following form:
with non-negative initial conditions S(0) = S0 > 0, E(0) = E0 > 0, I(0) = I0 > 0, R(0) = R0 ≥ 0. All parameters are defined as contact rates between individual categories.
Symbol | Biological definition |
S(0) | Initial susceptible individuals |
E(0) | Initial exposed individuals |
I(0) | Initial symptomatic infected individuals |
R(0) | Initial recovered individuals |
β | Infection rate |
Λ | Recruitment rate |
k | Transition rate of exposed individuals to the infected class |
α | Transition rate of exposed individuals to the recovered class |
γ | Natural recovery rate |
µ | Natural death rate |
Infectious disease models cannot be well understood only by biological techniques. Model analysis techniques and computational simulations can serve a great role in predicting and analyzing the model dynamics and identifying critical model parameters. Recently, some techniques of model analysis have been applied such as sensitivity and elasticity methods. They are useful tools to determine which variable or parameter is sensitive to a specific condition [12],[17],[18]. Calculating the basic reproduction numbers at the equilibrium points can also provide a great environment to discuss infectious disease models more widely and accurately [13],[14]. Here, in this study, we focus on three important techniques of model analysis: basic reproduction numbers, local sensitivity and elasticity methods. More details about the suggested techniques are discussed in the following sections.
In epidemiology, the next-generation matrix is used to derive the basic reproduction number for a compartmental model of the spread of infectious disease. This method was proposed by Diekmann et al. (1990) [35] and van den Driessche and Watmough (2002) [36]. One of the most important parameters for epidemiological models is the basic reproduction number [37]. Using Equation 2, we can easily derive R0 at an equilibrium point E0. The suggested model here includes two infected states, E and I, and two uninfected states, S and R. Let X be a vector of infected classes and Y be a vector of uninfected classes. They are shown below:
To find equilibrium points for the model, let us set the right-hand side of Equation 2 equal to zero. Then, the equilibrium points are obtained. The first equilibrium is the COVID-19-free equilibrium point, which is represented by
The second type of equilibrium is called the endemic equilibrium point. It is given below:
where
Using the next generation method, we have the vector functions
Then, the Jacobian matrices for M, G at E0 are given below:
Since W is a non-singular matrix, W−1 can be calculated:
Therefore, the basic reproduction number is the spectral radius of HW−1. Thus, the basic reproduction number for Equation 2 is
The concept of elasticity for infectious disease models has an effective role in determining the sensitivity between R0 and the model parameters. For calculating such coefficients, the following main formula is used:
where ω is the set of all parameters. Using Equation 5, we can calculate the elasticity index of R0 for each model parameter. They are given below:
Using the estimated parameters, we can compute the model elasticity; see Table 2.
Parameter | |
Λ | 1 |
k | 0.14039612 |
α | −0.403487536 |
γ | −0.9996225141 |
β | 1 |
µ | −1.0004274882 |
The results given in Table 2 show the positive and negative signs for the model elasticity. These give us the direct or indirect relations between R0 and the model parameters. For example, this coefficient is positive for the set of parameters {Λ, k, β}. This means that when these parameters, {Λ, k, β}, are increased, the value of R0 is also increased, and this virus spreads further. On the other hand, the signs of {γ, α, µ} are negative. This means that the value of the basic reproduction number can be reduced by increasing such parameters. Interestingly, β is the most significant positive parameter that can be utilized to reduce R0. For example, if the value of β is decreased by 10%, then the basic reproduction number will be reduced to R0 = 0.52886708. Furthermore, reducing the contact rate between individuals can impact the number of infected people from the disease.
The concept of local sensitivity analysis is another step forward for studying infection disease models and identifying the model critical elements. This is simply used for determining the sensitivity of each model species with respect to the model parameters. This method was recently considered in [14],[17],[18],[38]. Assume that there are p compartments (yi for i = 1,2,...,p) and q parameters (bj for j = 1,2,...,q) in an infectious disease model. The model balanced equations are represented by the following system of differential equations:
where
The full-normalization sensitivities are given as follows:
The half-normalization sensitivities are defined as follows:
The non-normalization sensitivities can be expressed as follows:
where
Computational techniques are effective tools and frequently required to analyze some complex infectious disease models. The estimated parameter values and initial populations are obtained from the real data in the Kurdistan Region of Iraq given in the Appendices. The model estimated parameters are α = 0.23, k = 0.34, γ = 0.08, β = 0.17, µ = 0.00003, Λ = 182.46, and the model initial variables are S0 = 6 × 106, E0 = 105.26, I0 = 1027, R0 = 1027. Using System Biology Toolbox (SBedit) in MATLAB, we determine the numerical approximate solutions of the model (Equation 2); see Figures 5 and 6. Numerical simulations are calculated in two-dimensional planes. All model computational results here are very interesting and provide some biological aspects. Firstly, it can be seen that Figure 5 shows the model dynamics of susceptible, exposed, asymptomatic infected and recovered people. We have noticed that the number of healthy people decreases gradually and becomes stable after 500 days, while the dynamics of recovered people increases and then becomes more flat after 500 days as well. Then, from 200 to 600 days, the numbers of exposed and infected individuals change dramatically. More interestingly, Figure 6 shows the comparison of total populations between the real data cases shown in Table 3 and the model equations given in Equation 2. For example, the total populations of infected people for the model equations and real data are given in Figure 6a. Then, Figure 6b shows the total populations of recovered individuals for model equations and real data. The results show good agreement between the real data and the model results. Finally, it is clear that our model results are fitted for the confirmed cases in Kurdistan. This means that the suggested model here can further be used to predict and give more control strategies.
Control strategies and preventions for the COVID-19 pandemic may not be well understood only by healthcare techniques and biological concepts. Mathematical models and computational simulations have been widely used for further improvements and identifying the model critical parameters. Therefore, identifying such parameters becomes an issue that can be further studied, and more techniques can be proposed. Sensitivity of model parameters helps international efforts to suggest more control strategies and preventions. Accordingly, the suggested SEIR model in this study plays an effective role in identifying the model critical elements using elasticity and sensitivity of R0. Thus, the model sensitivities were calculated using three different techniques: non normalizations, half normalizations, and full normalizations. The results showed that the most effective parameters for spreading this disease are contact rate among people, transition rate from exposed class to the infected class and natural recovery rate. Interestingly, identifying the model critical parameters here will help to suggest further interventions and control strategies in the Kurdistan Region of Iraq. The suggested SEIR model can be further developed and can have added more transmission rates between model states. For example, the vaccination compartment with its contact rates can be added to the model. This helps to study this disease more widely and accurately. Further techniques of model analysis such as optimal control will also be applied to the model to find more control strategies.
[1] |
Sebola MR, Mativeng PT, Pretorius J (2018) A Benchmark Study of Waste Tyre Recycling in South Africa to European Union Practice. Procedia CIRP, 25th CIRP Life Cycle Engineering (LCE) Conference: 69: 950–955. https://doi.org/10.1016/j.procir.2017.11.137 doi: 10.1016/j.procir.2017.11.137
![]() |
[2] |
Bockstal L, Berchem T, Schmetz Q, Richel A, et al. (2019) Devulcanization and reclaiming of tyres and rubber by physical and chemical processes: A review. J Clean Prod 236: 117574. https://doi.org/10.1016/j.jclepro.2019.07.049 doi: 10.1016/j.jclepro.2019.07.049
![]() |
[3] |
Siddika A, Mamun MAA, Alyousef R, et al. (2019) Properties and utilizations of waste tyre rubber in concrete: A review. Constr Build Mater 224: 711–731. https://doi.org/10.1016/j.conbuildmat.2019.07.108 doi: 10.1016/j.conbuildmat.2019.07.108
![]() |
[4] | Sattayanurak S, Sahakaro K, Kaewsakul W, et al. (2019) Utilization of Organoclay as Secondary Filler in Silica-reinforced Natural Rubber Tyre Tread Compounds. In: Fall 196th Technical Meeting of Rubber Division In press. |
[5] | Shulman VL (2019) Chapter 26 Tyre Recycling, In: Letcher TM, Vallero DA (Eds.), Waste, 2Eds., Academic Press, 489–515. https://doi.org/10.1016/B978-0-12-815060-3.00026-8 |
[6] |
Ramarad S, Khalid M, Ratnam CT, et al. (2015) Waste tyre rubber in polymer blends: A review on the evolution, properties and future. Prog Mater Sci 72:100–140. https://doi.org/10.1016/j.pmatsci.2015.02.004 doi: 10.1016/j.pmatsci.2015.02.004
![]() |
[7] |
Antoniou N, Zabaniotou A (2015) Experimental proof of concept for a sustainable End of Life Tyres pyrolysis with energy and porous materials production. J Clean Prod 101: 323–336. https://doi.org/10.1016/j.jclepro.2015.03.101 doi: 10.1016/j.jclepro.2015.03.101
![]() |
[8] |
Choi G-G, Jung S-H, Oh S-J, et al. (2014) Total utilization of waste tyre rubber through pyrolysis to obtain oils and CO2 activation of pyrolysis char. Fuel Process Technol 123: 57–64. https://doi.org/10.1016/j.fuproc.2014.02.007 doi: 10.1016/j.fuproc.2014.02.007
![]() |
[9] |
Hürdoğan E, Ozalp C, Kara O, et al. (2017) Experimental investigation on performance and emission characteristics of waste tyre pyrolysis oil–diesel blends in a diesel engine. Int J Hydrog Energy 42:23373–23378. https://doi.org/10.1016/j.ijhydene.2016.12.126 doi: 10.1016/j.ijhydene.2016.12.126
![]() |
[10] |
Martínez JD, Puy N, Murillo R, et al. (2013) Waste tyre pyrolysis–A review. Renew Sustain Energy Rev 23:179–213. https://doi.org/10.1016/j.rser.2013.02.038 doi: 10.1016/j.rser.2013.02.038
![]() |
[11] |
Martínez JD, Cardona-Uribe N, Murillo R, et al. (2019) Carbon black recovery from waste tyre pyrolysis by demineralization: Production and application in rubber compounding. Waste Manag 85: 574–584. https://doi.org/10.1016/j.wasman.2019.01.016 doi: 10.1016/j.wasman.2019.01.016
![]() |
[12] |
Chen D, Ling C, Wang T, et al. (2018) Prediction of tyre-pavement noise of porous asphalt mixture based on mixture surface texture level and distributions. Constr Build Mater 173: 801–810. https://doi.org/10.1016/j.conbuildmat.2018.04.062 doi: 10.1016/j.conbuildmat.2018.04.062
![]() |
[13] |
Bocca B, Forte G, Petrucci F, et al. (2009) Metals contained and leached from rubber granulates used in synthetic turf areas. Sci Total Environ 407: 2183–2190. https://doi:10.1016/j.scitotenv.2008.12.026 doi: 10.1016/j.scitotenv.2008.12.026
![]() |
[14] |
Yamaguchi K, Kinoshita T, Akita S (2006) Thermal treatment of waste tyre fly ash with polyvinyl chloride: Selective leaching of Zinc with water. Ind Eng Chem Res 45: 1211–1216. https://doi.org/10.1021/ie051118x doi: 10.1021/ie051118x
![]() |
[15] |
Zou C, Ren Y, Li S, et al. (2022) Effects of molten salt thermal treatment on the properties improvement of waste tyre pyrolytic char. Waste Manag 149: 53–59. https://doi.org/10.1016/j.wasman.2022.05.028 doi: 10.1016/j.wasman.2022.05.028
![]() |
[16] |
Jiang G, Pan J, Deng W, et al. (2022) Recovery of high pure pyrolytic carbon black from waste tyres by dual acid treatment. J Clean Product 374: 133893. https://doi.org/10.1016/j.jclepro.2022.133893 doi: 10.1016/j.jclepro.2022.133893
![]() |
[17] |
Bernardo M, Lapa N, Gonçalves M, et al. (2012) Study of the organic extraction and acidic leaching of chars obtained in the pyrolysis of plastics, tyre rubber and forestry biomass wastes. Proced Eng 42: 1739–1746. https://doi.org/10.1016/j.proeng.2012.07.567 doi: 10.1016/j.proeng.2012.07.567
![]() |
[18] | Levenspiel O (1972) Chemical Reaction Engineering, In: John Wiley and Sons, 3Eds., New York: 578. |
[19] |
Dube G, Osifo P, Rutto H (2014) Preparation of bagasse ash/CaO/ammonium acetate sorbent and modelling their desulphurization reaction. Clean Techn Environ Policy 16: 891–900. https://doi.org/10.1007/s10098-013-0681-8 doi: 10.1007/s10098-013-0681-8
![]() |
[20] |
Rutto H, Enweremad C (2012) Dissolution of a South African calcium based material using urea: An optimized process. Korean J Chem Eng 29: 1–8. https://doi.org/10.1007/s11814-011-0136-z doi: 10.1007/s11814-011-0136-z
![]() |
[21] |
He F, Ma B, Wang C, Zuo Y, et al. (2022) Dissolution behavior and porous kinetics of limonitic laterite during nitric acid atmospheric leaching. Minerals Eng 185: 107671. https://doi.org/10.1016/j.mineng.2022.107671 doi: 10.1016/j.mineng.2022.107671
![]() |
[22] |
Ajiboye EA, Panda PK, Adebayo AO, et al. (2019) Leaching kinetics of Cu, Ni and Zn from waste silica rich integrated circuits using mild nitric acid. Hydrometallurgy 188: 161–168. https://doi.org/10.1016/j.hydromet.2019.06.016 doi: 10.1016/j.hydromet.2019.06.016
![]() |
[23] |
Seng-eiad S, Jitkarnka S (2016) Untreated and HNO3-treated pyrolysis char as catalysts for pyrolysis of waste tyre: In-depth analysis of tyre-derived products and char characterization. J Anal Appl Pyrolysis 122: 151–159. https://doi.org/10.1016/j.jaap.2016.10.004 doi: 10.1016/j.jaap.2016.10.004
![]() |
[24] | Manoj B (2012) Chemical demineralization of high volatile Indian bituminous coal by carboxylic acid and characterization of the products by SEM/EDS. J Environ Res Dev 6: 653–659. http://archives.christuniversity.in/items/show/880 |
[25] |
Zhou J, Zhao J, Yang F, et al. (2020) Leaching kinetics of potassium and aluminum from phosphorus-potassium associated ore in HCl-CaF2 system. Sep Purif Technol 253: 117528. https://doi.org/10.1016/j.seppur.2020.117528 doi: 10.1016/j.seppur.2020.117528
![]() |
[26] |
Koech L, Everson R, Neomagus H, et al. (2015) Leaching kinetics of bottom ash waste as a source of calcium ions. J Air Waste Manag Assoc 65: 126–132. https://doi.org/10.1080/10962247.2014.978958 doi: 10.1080/10962247.2014.978958
![]() |
[27] |
Koech L, Rutto H, Everson R, et al. (2014) Semi-empirical model for limestone dissolution in adipic acid for wet flue gas desulfurization. Chem Eng Technol 37: 1919–1928. https://doi.org/10.1002/ceat.201400248 doi: 10.1002/ceat.201400248
![]() |
[28] |
Raza N, Zafar ZI, Najam-ul-Haq (2013) An analytical model approach for the dissolution kinetics of magnesite ore using ascorbic acid as leaching agent. Int J Met 1: 352496. https://doi.org/10.1155/2013/352496 doi: 10.1155/2013/352496
![]() |
[29] |
Cao R, Jia Z, Zhang Z, et al. (2020) Leaching kinetics and reactivity evaluation of ferronickel slag in alkaline conditions. Cem Concr Res 137: 106202. https://doi.org/10.1016/j.cemconres.2020.106202 doi: 10.1016/j.cemconres.2020.106202
![]() |
[30] |
Sharma S, Agarwal GK, Dutta NN (2020) Kinetic study on leaching of Zn and Cu from spent low-temperature shift catalyst (CuO/ZnO/Al2O3): application of taguchi design. J Mater Cycles Waste Manag 22: 1509–1520. https://doi.org/10.1007/s10163-020-01038-x doi: 10.1007/s10163-020-01038-x
![]() |
[31] |
He J, Yang J, Tariq SM, et al (2020) Comparative investigation on copper leaching efficiency from waste mobile phones using various types of ionic liquids. J Clean Product 256: 120368. https://doi.org/10.1016/j.jclepro.2020.120368 doi: 10.1016/j.jclepro.2020.120368
![]() |
[32] |
Rozmysłowska-Wojciechowska A, Mitrzak J, Szuplewska A, et al. (2020) Engineering of 2D Ti3C2 MXene surface charge and its influence on biological properties. Materials 10: 2347. https://doi.org/10.3390/ma13102347 doi: 10.3390/ma13102347
![]() |
[33] |
Kumar A, Choudhary R, Kumar A (2021) Aging characteristics of asphalt binders modified with waste tire and plastic pyrolytic chars. PLoS One 19: 16. https://doi.org/10.1371/journal.pone.0256030 doi: 10.1371/journal.pone.0256030
![]() |
[34] |
Czarna-Juszkiewicz D, Kunecki P, Cader J, et al. (2023) Review in waste tire management—potential applications in mitigating environmental pollution. Materials 16: 5771. https://doi.org/10.3390/ma16175771 doi: 10.3390/ma16175771
![]() |
[35] |
Li S, Tran TQ, Li Q, et al. (2023) Zn leaching recovery and mechanisms from end-of-life tire rubber. Resour Conserv Recycl 194: 107004. https://doi.org/10.1016/j.resconrec.2023.107004 doi: 10.1016/j.resconrec.2023.107004
![]() |
[36] |
Undri A, Sacchi B, Cantisani E, et al. (2013) Carbon from microwave assisted pyrolysis of waste tires. J Anal Appl Pyrol 104: 396–404. https://doi.org/10.1016/j.jaap.2013.06.006 doi: 10.1016/j.jaap.2013.06.006
![]() |
[37] |
Battsengel A, Batnasan A, Narankhuu A, et al. (2018) Recovery of light and heavy rare earth elements from apatite ore using sulphuric acid leaching, solvent extraction and precipitation. Hydrometallurgy 179: 100–109. https://doi:10.1016/j.hydromet.2018.05.024 doi: 10.1016/j.hydromet.2018.05.024
![]() |
[38] |
Faraji F, Alizadeh A, Rashchi F, et al. (2022) Kinetics of leaching: A review. Rev Chem Eng 38: 113–148. https://doi.org/10.1515/revce-2019-0073 doi: 10.1515/revce-2019-0073
![]() |
1. | Pierluigi Colli, Gianni Gilardi, Jürgen Sprekels, Asymptotic analysis of a tumor growth model with fractional operators, 2020, 120, 18758576, 41, 10.3233/ASY-191578 | |
2. | Pierluigi Colli, Gianni Gilardi, Jürgen Sprekels, A Distributed Control Problem for a Fractional Tumor Growth Model, 2019, 7, 2227-7390, 792, 10.3390/math7090792 | |
3. | Jinlong Wei, Jinqiao Duan, Guangying Lv, Kinetic Solutions for Nonlocal Scalar Conservation Laws, 2018, 50, 0036-1410, 1521, 10.1137/16M108687X | |
4. | Gerardo Huaroto, Wladimir Neves, Solvability of the fractional hyperbolic Keller–Segel system, 2023, 74, 14681218, 103957, 10.1016/j.nonrwa.2023.103957 |
Symbol | Biological definition |
S(0) | Initial susceptible individuals |
E(0) | Initial exposed individuals |
I(0) | Initial symptomatic infected individuals |
R(0) | Initial recovered individuals |
β | Infection rate |
Λ | Recruitment rate |
k | Transition rate of exposed individuals to the infected class |
α | Transition rate of exposed individuals to the recovered class |
γ | Natural recovery rate |
µ | Natural death rate |
Parameter | |
Λ | 1 |
k | 0.14039612 |
α | −0.403487536 |
γ | −0.9996225141 |
β | 1 |
µ | −1.0004274882 |
Symbol | Biological definition |
S(0) | Initial susceptible individuals |
E(0) | Initial exposed individuals |
I(0) | Initial symptomatic infected individuals |
R(0) | Initial recovered individuals |
β | Infection rate |
Λ | Recruitment rate |
k | Transition rate of exposed individuals to the infected class |
α | Transition rate of exposed individuals to the recovered class |
γ | Natural recovery rate |
µ | Natural death rate |
Parameter | |
Λ | 1 |
k | 0.14039612 |
α | −0.403487536 |
γ | −0.9996225141 |
β | 1 |
µ | −1.0004274882 |