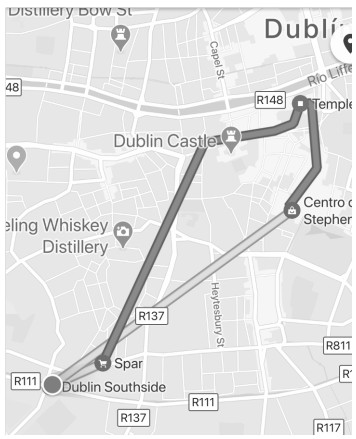
Type 2 diabetes (T2D) is characterized by a state of hyperglycemia in the blood due to insulin resistance developed by organs such as muscle, liver, and adipose tissue. A common factor in individuals with T2D is mitochondrial dysfunction. Mitochondria are dynamic organelles responsible for energy and antioxidant metabolism in the cells. Estrogens, such as 17β-estradiol (E2), are steroid hormones that have shown a great capacity to regulate mitochondrial function and dynamics through estrogen receptors (ERs), modulating the expression of mitochondrial biogenesis-related genes and cell signaling mechanisms. The accumulation of reactive oxygen species, the low capacity for ATP synthesis, and morphological alterations are some of the mitochondrial processes impaired in T2D. Insulin signaling and secretion by pancreatic β-cells, ATP-dependent processes, are also altered in T2D. In this review, mitochondria were exposed as the central axis for the action of estrogens in individuals with T2D. Estrogens increased glucose uptake, insulin signaling, and mitochondrial bioenergetics, and decreased ectopic lipid accumulation in non-adipose tissues and oxidative stress, among other processes, in various preclinical and clinical models of diabetes. The development of strategies to target compounds to mitochondria could represent a novel therapeutic alternative to potentiate the effects of estrogens on this organelle in patients with insulin resistance and T2D.
Citation: Geovanni Alberto Ruiz-Romero, Carolina Álvarez-Delgado. Effects of estrogens in mitochondria: An approach to type 2 diabetes[J]. AIMS Molecular Science, 2024, 11(1): 72-98. doi: 10.3934/molsci.2024006
[1] | Farah Liyana Azizan, Saratha Sathasivam, Nurshazneem Roslan, Ahmad Deedat Ibrahim . Logic mining with hybridized 3-satisfiability fuzzy logic and harmony search algorithm in Hopfield neural network for Covid-19 death cases. AIMS Mathematics, 2024, 9(2): 3150-3173. doi: 10.3934/math.2024153 |
[2] | Nadiyah Hussain Alharthi, Mdi Begum Jeelani . Analyzing a SEIR-Type mathematical model of SARS-COVID-19 using piecewise fractional order operators. AIMS Mathematics, 2023, 8(11): 27009-27032. doi: 10.3934/math.20231382 |
[3] | Said Gounane, Jamal Bakkas, Mohamed Hanine, Gyu Sang Choi, Imran Ashraf . Generalized logistic model with time-varying parameters to analyze COVID-19 outbreak data. AIMS Mathematics, 2024, 9(7): 18589-18607. doi: 10.3934/math.2024905 |
[4] | Mohammad Hamidi, Florentin Smarandache . Valued-inverse Dombi neutrosophic graph and application. AIMS Mathematics, 2023, 8(11): 26614-26631. doi: 10.3934/math.20231361 |
[5] | Deniz UÇAR, Elçin ÇELİK . Analysis of Covid 19 disease with SIR model and Taylor matrix method. AIMS Mathematics, 2022, 7(6): 11188-11200. doi: 10.3934/math.2022626 |
[6] | Xiaoying Pan, Longkun Tang . A new model for COVID-19 in the post-pandemic era. AIMS Mathematics, 2024, 9(8): 21255-21272. doi: 10.3934/math.20241032 |
[7] | Tahir Khan, Fathalla A. Rihan, Muhammad Bilal Riaz, Mohamed Altanji, Abdullah A. Zaagan, Hijaz Ahmad . Stochastic epidemic model for the dynamics of novel coronavirus transmission. AIMS Mathematics, 2024, 9(5): 12433-12457. doi: 10.3934/math.2024608 |
[8] | M. J. Huntul . Inverse source problems for multi-parameter space-time fractional differential equations with bi-fractional Laplacian operators. AIMS Mathematics, 2024, 9(11): 32734-32756. doi: 10.3934/math.20241566 |
[9] | Ishtiaq Ali, Sami Ullah Khan . Dynamics and simulations of stochastic COVID-19 epidemic model using Legendre spectral collocation method. AIMS Mathematics, 2023, 8(2): 4220-4236. doi: 10.3934/math.2023210 |
[10] | CW Chukwu, S. Y. Tchoumi, Z. Chazuka, M. L. Juga, G. Obaido . Assessing the impact of human behavior towards preventative measures on COVID-19 dynamics for Gauteng, South Africa: a simulation and forecasting approach. AIMS Mathematics, 2024, 9(5): 10511-10535. doi: 10.3934/math.2024514 |
Type 2 diabetes (T2D) is characterized by a state of hyperglycemia in the blood due to insulin resistance developed by organs such as muscle, liver, and adipose tissue. A common factor in individuals with T2D is mitochondrial dysfunction. Mitochondria are dynamic organelles responsible for energy and antioxidant metabolism in the cells. Estrogens, such as 17β-estradiol (E2), are steroid hormones that have shown a great capacity to regulate mitochondrial function and dynamics through estrogen receptors (ERs), modulating the expression of mitochondrial biogenesis-related genes and cell signaling mechanisms. The accumulation of reactive oxygen species, the low capacity for ATP synthesis, and morphological alterations are some of the mitochondrial processes impaired in T2D. Insulin signaling and secretion by pancreatic β-cells, ATP-dependent processes, are also altered in T2D. In this review, mitochondria were exposed as the central axis for the action of estrogens in individuals with T2D. Estrogens increased glucose uptake, insulin signaling, and mitochondrial bioenergetics, and decreased ectopic lipid accumulation in non-adipose tissues and oxidative stress, among other processes, in various preclinical and clinical models of diabetes. The development of strategies to target compounds to mitochondria could represent a novel therapeutic alternative to potentiate the effects of estrogens on this organelle in patients with insulin resistance and T2D.
In the mid-19th century, the English physician John Snow [9] refused to accept the theories of cholera infection prevailing at that time. Both the sharing of clothing and transmission through the air seemed to him to be insufficient explanations for what was happening in London, under an epidemic that was undermining the entire population of any social class. He established a famous practical example of spatial epidemiology, measuring the distances of each patient to the nearest water source.
Not only Covid-19 [11,22], but many other infectious diseases [6] are forcing us, in general, to rethink new techniques for describing and discovering spatial epidemiology, that is, "the description and analysis of geographic variations in disease with respect to demographic, environmental, behavioral, socioeconomic, genetic, and infectious risk factors" [10]. To this end, we can draw on data that reflect human behavior in terms of spatial location and movement. In particular, geolocation data [24], which includes geographical location information associated with the hosting device (e.g., mobile phone), is key for modeling the spread and evolution of infectious diseases in space and time.
Using this kind of data, we cannot only track the movement patterns of individuals, but also derive general mobility patterns for the population over time. They give insight into, e.g., "the prediction of future moves, detecting modes of transport, mining trajectory patterns and recognizing location-based activities" [13]. That is, geolocation data is key data computational spatial empidemiologic models and simulations.
The idea that a microscopic coronavirus in the city of Wuhan has caused an economic crisis in Europe reminds us of Edward Lorenz's famous speech at MIT, where he raised how the flapping of a butterfly in Brazil could cause a tornado in Texas. That is, the well-known butterfly effect and its repercussions on chaos theory.
In this work, data about spatial population movement such as geolocation data are used as key sources of insight in the way infectious diseases spread in space and time among individuals. To this end, this work proposes a mathematical foundation following an inverse methodology on the chaotic bases [18] since it raises the search of the starting attractor or "patient zero". This procedure collects the ideas of reverse engineering, as a way of establishing the unknown initial parameters of a problem whose solutions and effects are known.
In particular, tracking and analyzing population movements are key to get insights about patterns of propagation of environmentally-transmitted pathogens. For example in the case of Covid-19, "[...] changing geography of migration, the diversification of jobs taken by migrants, the rapid growth of tourism and business trips, and the longer distance taken by people for family reunion are what make the spread of COVID-19 so differently from that of SARS" [19].
To this end, we introduce a graph-based approach to model population movements in space and time, including a health status of individuals that allows us to classify risks for individuals as well as spatial locations and temporal periods.
The main methods to be used will be differential geometry of curves adapted to the symptomatology of the disease and graph theory for its representation and monitoring [16]. In its inverse reasoning, we can establish algorithms that, e.g., facilitate finding a "patient zero", classify possible originators of the contagion [1,3,12,17,20], or classify spatial locations with respect to the spread of pathogens.
As a key source of human mobility patterns, we consider mobile phone location data. The high penetration of mobile phones in the population allows a very representative survey of mobility dynamics [14].
The trajectories on which we base the monitoring of population movements are those recorded by the smartphones devices with GPS. We know that both smartphones and social networks [23] have the ability to store the paths followed by their owners, just as telephone companies can track that path followed by their customers [7,8]. This information is collected both by these devices and by the telephone companies, and can be represented geospatially by the different navigation systems. As an example we can see the path followed by an smartphone and represented in Figure 1 using Google Maps software:
Definition 2.1. For every patient P we distinguish three disjointed and sequential time intervals (as can be seen in the medical graphs in Figure 2) that we will note by:
1. H: where the person is "healthy". In the timeline it will be distinguished by a simple line and during this interval [5] (before infection) the value of the state variable will be 1.
2. C: where the person is "contagious" (incubation period). This interval or latency period will be estimated, given the patient's symptoms, based on the maximum and minimum time it could take to become "ill". It will be represented by a double line and its state variable will be 2. Currently this period is from 0 to 7 days aproximately.
3. I: where the patient is "ill". It will be represented by a triple line, together with the previous one completes the incubation time [21] and its state variable will be 3.
Definition 2.2. To establish the path we use the notation of differential geometry by using Cartesian coordinates, incorporating the state variable over time ρ(t)∈{1,2,3} remaining:
→α(t)=(x(t),y(t),ρ(t))witht∈{H∪C∪I} |
and whose graph is represented in Figure 3.
Definition 2.3. We will say that a contagion has occurred if, given two patients Pi and Pj it is fulfilled:
● the paths cross in a sufficiently close environment, i.e. there is a maximum transmission distance M such that:
foraninstanttitisfulfilledthat√(xi(t)−xj(t))2+(yi(t)−yj(t))2≤M |
To simplify the calculation, without loss of generality, we will consider that this distance M is null, or what is the same, they are in the same place. With this we have:
foraninstanttitisfulfilledthat(xi(t),yi(t))=(xj(t),yj(t)) |
● one patient's status must be "contagious" and the other patient's status must be "ill", i.e., t∈C for Pi and t∈I for Pj or vice versa. This is simplified as ρi(t)⋅ρj(t)=6 (product of a state that is "contagious" by another "ill").
We will note the contagion between both patients as Pi∼Pj.
Notation 2.4. The point of infection of each patient P will be identified with the symbol ⊗ and its coordinates will be →⊗=(x,y,n,t) where:
● x and y are the cartesian coordinates of the place of infection
● n is the variable where the number of infections produced in this place is accumulated.
● t is the variable that indicates the furthest moment in which the contagion occurred in this place.
It can be seen in the contagion graph of Figure 4 that a different situation occurs at each confluence ⊗i since in
⊗1 Both patients are healthy
⊗2 The second patient P2 has been infected by the first P1
⊗3 The first patient P1 is still "healthy" after meeting the second P2 who is ill. They may not have coincided in time at this intersection and the first one was not in a "contagious" state.
⊗4 Here both patients are already "ill"
Procedure 3.1 (Intersections analysis). Given two different paths, →αi and →αj, these can be subdivided into segments, being considered therefore as polygonal *. Hence, the analysis of intersections is restricted to the calculation of common points between two segments of different paths [16]. Let us consider the segments between points:
*A polygonal sufficiently close to the curve is considered. Its existence is assured by Schoenflies theorem for polygonal Jordan curves. This concept also appears in the formulation of the length of a parametric curve, making use of the approximations by sufficiently small segments and its use in the Riemann integral.
Sip=¯(xip(t1),yip(t1))(xip+1(t2),yip+1(t2))∈→αi |
Sjq=¯(xjq(t3),yjq(t3))(xjq+1(t4),yjq+1(t4))∈→αj |
1. We check for the existence of temporal (one-dimensional) intersection, that is, time concurrence:
(t1,t2)∩(t3,t4)≠Ø⇔t1≤t4∧t3≤t2 |
2. We check for geometric (two-dimensional) intersection:
(a) It can be done by solving the system, as long as it is well-conditioned:
{xip(t1)+λ(xip+1(t2)−xip(t1))=xjq(t3)+μ(xjq+1(t4)−xjq(t3))yip(t1)+λ(yip+1(t2)−yip(t1))=yjq(t3)+μ(yjq+1(t4)−yjq(t3)) |
If there are 0≤λ≤1 and 0≤μ≤1 that fulfill both equations or are collinear, that is:
|xip+1(t2)−xip(t1)xjq+1(t4)−xjq(t3)yip+1(t2)−yip(t1)yjq+1(t4)−yjq(t3)|=0 |
where contagion could occur if they are coincidental:
xip+1(t2)−xip(t1)yip+1(t2)−yip(t1)=xjq+1(t4)−xjq(t3)yjq+1(t4)−yjq(t3)=xip(t1)−xjq(t3)yip(t1)−yjq(t3) |
In any other situation, as there are no points in common between the segments, the minimum distances between each point and the opposite segment are calculated. Finally, it is checked whether the minimum of them is below the maximum transmission distance, so that there is a possibility of contagion (as Figure 5):
min{d1,d2,d3,d4}≤M |
In this case the execution time for n segments would be
Θ(n2) |
(b) Or using Bentley's & Ottmann algorithm [4], whose execution time for n segments with s points of intersection is
Θ(nlog(n)+slog(n)) |
Procedure 3.2 (Establishing Contagion). The big data algorithm must fulfill the following phases:
1. Analyze the intersections of patient trajectories by pairs (→αi and →αj) where contagion may occur [15]:
findtsuchthat(xi(t),yi(t))=(xj(t),yj(t))withρi(t)⋅ρj(t)=6 |
2. In each analysis, establish the possible point of contagion (if any) and increase both its contagion index and its most remote time variable in which a contagion occurred
For→⊗=(x,y,n,t),isexecutedn=n+1 |
3. Patients are classified according to the sum of the indexes of all the points of contagion contained in their trajectory. In this way, we will order the patients from the one that has caused the most infections to the one that has caused the least.
4. Patients are classified according to the lowest value of t contained in their points of contagion. With this we can establish patient zero.
With this procedure, we calculate the distribution of contagion points of known patients →⊗k, and the final number of contagions in each one of them →⊗k, which gives us an image of the city as shown in Figure 6:
Once the possible cut-off points between known patient routes have been established, we ask ourselves whether there are any undetected patients who can be deduced from the possible points of contagion. This question involves a probabilistic analysis of what the possible path of the unknown patient would look like.
Let us suppose that there is an unknown patient that has been causing an uncontrolled contagion, which we will call Px.
1. If the contagion has occurred at an intersection →⊗ij of two "contagious" patients Pi and Pj, that is, ρi(t)⋅ρj(t)=4, it could be that:
(a) one of them was already really ill: This forces a recalculation of the C and I intervals for both. In this case, if the one who was already ill had been Pi, he must have been previously infected by a stranger, and therefore there was a cross with Px. Similarly for Pj.
(b) neither of them infected the other: In this case there is a coincidence of the path of both with the one of Px at the point of infection.
In both cases we assign a new contagion in the point →⊗ij, although in the first assumption we also know that there is some point in the path of Pi or Pj in the state C where it must have crossed with that of Px in the state I. Therefore, if we call t(→⊗ij,C0i) and t(→⊗ij,C0j) the time from the crossing point to the place where Pi and Pj met Px respectively, which are C0i and C0j, then the crossing probabilities (CP) with Px of each interval are set as:
PC(x,i)=t(→⊗ij,C0i)[Ci] |
PC(x,j)=t(→⊗ij,C0j)[Cj] |
where [Ci] and [Cj] are the times at which Pi and Pj have been contagious.
2. If the infection has occurred in the C range of any patient Pi, the path of Px matches that of Pi with ρi(t)=2 and ρx(t)=3. In this case it is known that there is some point in the path of Pi in the state C where it should have crossed with that of Px in the state I. Here the probability of the whole C interval is equal to 1.
The zone graph, in these cases, is represented by Figure 7
Procedure 4.1 (Inverse graphs by big data). The big data algorithm will then be based on finding, of all existing paths of citizens that go through this area, those that achieve a higher sum of probabilities at the intersections of the N intervals of known contagion [12].
1. To do this, the route followed by each citizen's smartphone Px is analysed and its intersections with the probable paths, assuming that this citizen was permanently ill:
→αx(t)=(x(t),y(t),3) |
2. This would cause a contagion at each intersection and would add probabilities to its final value.
N∑i=1PC(x,i)conPx∼Pi |
3. Those candidates with the highest score will be studied to see if they are carriers or not. A new set of patients will be deducted from the patients prior to the existing ones. Using the procedure recursively, we will reach patient zero.
After the theoretical development of the Inverse Graph method, the general geolocation algorithm has been programmed using a Computer Algebra System (CAS) as MatLab †. The developed algorithm has a quadratic execution time, i.e. Θ(n2) and its pseudo-code ‡ would be as follows:
†Algorithms and functions programmed in MatLab version R2020b and tested on a MacBook with processor 1.3 GHz Intel Core m7
‡All that appears between braces are comments
begin Read kml compress files from Google Maps timeline for each
user i. Build matrix Mi of coordinates and its convex hull.
Analyzes only disjunct concentrations of convex hulls (Figure 8)
M=[]; for i:1:users do M=[M;Mi] end for
Times = intervals(M); {Build matrix time interval for all users}
I = sort(Times) {Sort the intervals as a function of time}
point = []; inter = size(I, 1);
for j = 2:inter
for i = 1:j-1 {Check the space-time match}
if fit(Times(i), Times(j))
point = cuts(I, Times, M); {Found intersection points}
end if
end for
end for
end
Next Table 1 and Figures below (Figures 9 and 10) show execution times (in seconds) and the number of contact points that occurred between different people, based on user graph intersections for the last twelve days, with an average of 388 vertexes at their polygonal paths for each user
Users | Time | Points |
10 | 0.29 | 837 |
20 | 0.41 | 1755 |
30 | 0.72 | 2565 |
40 | 0.94 | 3595 |
50 | 1.20 | 4954 |
100 | 3.60 | 10746 |
150 | 7.68 | 15397 |
The capacity of Big Data to solve the problem will depend on the reduction that is made on the population to be analyzed for the search of the patient zero [20].
This reduction is conditioned by the need for temporal and geometric coincidence of the routes with those of the probable intervals. Therefore, it is a priority to establish the condition of temporal coincidence, which will considerably reduce the set of candidates (Figure 11), and then analyze the intersections in the plane.
Each element aij of a dispersed matrix of contagions A=(aij) would represent with values 0 or 1 the possibility that the patient Pi has infected the patient Pj, with i,j∈{1,…,N}
With this representation the rows i, whose sum of terms is greater N∑j=1aij, will correspond to the index of the most dangerous patients. The number of infections of the most dangerous patient will be indicated by
||A||∞=maxiN∑j=1aij |
The application of Markov Chains on arrays, whose elements represent the mesh of an area, will allow us to see to which position each individual moves in a unit of time and to which state of health he can evolve [3,17].
Neural networks can provide a trained system for the pre-selection of possible candidates for asymptomatic or unknown patients [1].
We thank the support of this paper from University of Málaga and CBUA (funding for open access charge: Universidad de Málaga / CBUA) and we thank also the anonymous reviewers whose suggestions helped improve and clarify this manuscript.. This research did not receive any specific grant from funding agencies in the public, commercial, or not-for-profit sectors.
The authors declare that there are no conflicts of interest.
[1] |
DeFronzo RA, Ferrannini E, Groop L, et al. (2015) Type 2 diabetes mellitus. Nat Rev Dis Primers 1: 15019. https://doi.org/10.1038/nrdp.2015.19 ![]() |
[2] |
Ley SH, Hamdy O, Mohan V, et al. (2014) Prevention and management of type 2 diabetes: Dietary components and nutritional strategies. Lancet 383: 1999-2007. https://doi.org/10.1016/S0140-6736(14)60613-9 ![]() |
[3] | Federation ID (2021) IDF diabetes atlas. Brussels, Belgium: International Diabetes Federation. Available from: https://diabetesatlas.org/. |
[4] |
Pinti MV, Fink GK, Hathaway QA, et al. (2019) Mitochondrial dysfunction in type 2 diabetes mellitus: an organ-based analysis. Am J Physiol Endocrinol Metab 316: E268-E285. https://doi.org/10.1152/ajpendo.00314.2018 ![]() |
[5] |
Duranova H, Valkova V, Knazicka Z, et al. (2020) Mitochondria: A worthwhile object for ultrastructural qualitative characterization and quantification of cells at physiological and pathophysiological states using conventional transmission electron microscopy. Acta Histochem 122: 151646. https://doi.org/10.1016/j.acthis.2020.151646 ![]() |
[6] |
Spinelli JB, Haigis MC (2018) The multifaceted contributions of mitochondria to cellular metabolism. Nat Cell Biol 20: 745-754. https://doi.org/10.1038/s41556-018-0124-1 ![]() |
[7] |
Larsen S, Nielsen J, Hansen CN, et al. (2012) Biomarkers of mitochondrial content in skeletal muscle of healthy young human subjects. J Physiol 590: 3349-3360. https://doi.org/10.1113/jphysiol.2012.230185 ![]() |
[8] |
Lin CC, Cheng TL, Tsai WH, et al. (2012) Loss of the respiratory enzyme citrate synthase directly links the Warburg effect to tumor malignancy. Sci Rep 2: 785. https://doi.org/10.1038/srep00785 ![]() |
[9] |
Sazanov LA (2015) A giant molecular proton pump: Structure and mechanism of respiratory complex I. Nat Rev Mol Cell Biol 16: 375-388. https://doi.org/10.1038/nrm3997 ![]() |
[10] |
Al Rasheed MRH, Tarjan G (2018) Succinate dehydrogenase complex: An updated review. Arch Pathol Lab Med 142: 1564-1570. https://doi.org/10.5858/arpa.2017-0285-RS ![]() |
[11] |
Neupane P, Bhuju S, Thapa N, et al. (2019) ATP synthase: Structure, function and inhibition. Biomol Concepts 10: 1-10. https://doi.org/10.1515/bmc-2019-0001 ![]() |
[12] |
Prasun P (2020) Role of mitochondria in pathogenesis of type 2 diabetes mellitus. J Diabetes Metab Disord 19: 2017-2022. https://doi.org/10.1007/s40200-020-00679-x ![]() |
[13] |
Russell OM, Gorman GS, Lightowlers RN, et al. (2020) Mitochondrial diseases: Hope for the Future. Cell 181: 168-188. https://doi.org/10.1016/j.cell.2020.02.051 ![]() |
[14] |
Miller WL, Auchus RJ (2011) The molecular biology, biochemistry, and physiology of human steroidogenesis and its disorders. Endocr Rev 32: 81-151. https://doi.org/10.1210/er.2010-0013 ![]() |
[15] |
Dard L, Blanchard W, Hubert C, et al. (2020) Mitochondrial functions and rare diseases. Mol Aspects Med 71: 100842. https://doi.org/10.1016/j.mam.2019.100842 ![]() |
[16] |
Dong H, Tsai SY (2023) Mitochondrial properties in skeletal muscle fiber. Cells 12: 2183. https://doi.org/10.3390/cells12172183 ![]() |
[17] |
Nunnari J, Suomalainen A (2012) Mitochondria: In sickness and in health. Cell 148: 1145-1159. https://doi.org/10.1016/j.cell.2012.02.035 ![]() |
[18] |
Popov LD (2020) Mitochondrial biogenesis: An update. J Cell Mol Med 24: 4892-4899. https://doi.org/10.1111/jcmm.15194 ![]() |
[19] |
Barshad G, Marom S, Cohen T, et al. (2018) Mitochondrial DNA transcription and its regulation: An evolutionary perspective. Trends Genet 34: 682-692. https://doi.org/10.1016/j.tig.2018.05.009 ![]() |
[20] |
Wang F, Zhang D, Zhang D, et al. (2021) Mitochondrial protein translation: Emerging roles and clinical significance in disease. Front Cell Dev Biol 9: 675465. https://doi.org/10.3389/fcell.2021.675465 ![]() |
[21] |
Demishtein-Zohary K, Azem A (2017) The TIM23 mitochondrial protein import complex: Function and dysfunction. Cell Tissue Res 367: 33-41. https://doi.org/10.1007/s00441-016-2486-7 ![]() |
[22] |
Palikaras K, Lionaki E, Tavernarakis N (2015) Balancing mitochondrial biogenesis and mitophagy to maintain energy metabolism homeostasis. Cell Death Differ 22: 1399-1401. https://doi.org/10.1038/cdd.2015.86 ![]() |
[23] |
Ding Q, Qi Y, Tsang SY (2021) Mitochondrial biogenesis, mitochondrial dynamics, and mitophagy in the maturation of cardiomyocytes. Cells 10: 2463. https://doi.org/10.3390/cells10092463 ![]() |
[24] |
Zhang B, Pan C, Feng C, et al. (2022) Role of mitochondrial reactive oxygen species in homeostasis regulation. Redox Rep 27: 45-52. https://doi.org/10.1080/13510002.2022.2046423 ![]() |
[25] |
Schieber M, Chandel NS (2014) ROS function in redox signaling and oxidative stress. Curr Biol 24: R453-R462. https://doi.org/10.1016/j.cub.2014.03.034 ![]() |
[26] |
Cox AG, Winterbourn CC, Hampton MB (2009) Mitochondrial peroxiredoxin involvement in antioxidant defence and redox signalling. Biochem J 425: 313-325. https://doi.org/10.1042/BJ20091541 ![]() |
[27] |
Ristow M, Schmeisser K (2014) Mitohormesis: Promoting health and lifespan by increased levels of reactive oxygen species (ROS). Dose Response 12: 288-341. https://doi.org/10.2203/dose-response.13-035.Ristow ![]() |
[28] |
Zarse K, Schmeisser S, Groth M, et al. (2012) Impaired insulin/IGF1 signaling extends life span by promoting mitochondrial L-proline catabolism to induce a transient ROS signal. Cell Metab 15: 451-465. https://doi.org/10.1016/j.cmet.2012.02.013 ![]() |
[29] |
Rovira-Llopis S, Banuls C, Diaz-Morales N, et al. (2017) Mitochondrial dynamics in type 2 diabetes: Pathophysiological implications. Redox Biol 11: 637-645. https://doi.org/10.1016/j.redox.2017.01.013 ![]() |
[30] |
de Brito OM, Scorrano L (2008) Mitofusin 2 tethers endoplasmic reticulum to mitochondria. Nature 456: 605-610. https://doi.org/10.1038/nature07534 ![]() |
[31] |
Tubbs E, Theurey P, Vial G, et al. (2014) Mitochondria-associated endoplasmic reticulum membrane (MAM) integrity is required for insulin signaling and is implicated in hepatic insulin resistance. Diabetes 63: 3279-3294. https://doi.org/10.2337/db13-1751 ![]() |
[32] |
Head B, Griparic L, Amiri M, et al. (2009) Inducible proteolytic inactivation of OPA1 mediated by the OMA1 protease in mammalian cells. J Cell Biol 187: 959-966. https://doi.org/10.1083/jcb.200906083 ![]() |
[33] |
Consolato F, Maltecca F, Tulli S, et al. (2018) m-AAA and i-AAA complexes coordinate to regulate OMA1, the stress-activated supervisor of mitochondrial dynamics. J Cell Sci 131: jcs213546. https://doi.org/10.1242/jcs.213546 ![]() |
[34] | Nan J, Nan C, Ye J, et al. (2019) EGCG protects cardiomyocytes against hypoxia-reperfusion injury through inhibition of OMA1 activation. J Cell Sci 132: jcs220871. https://doi.org/10.1242/jcs.220871 |
[35] |
Otera H, Mihara K (2011) Molecular mechanisms and physiologic functions of mitochondrial dynamics. J Biochem 149: 241-251. https://doi.org/10.1093/jb/mvr002 ![]() |
[36] |
Loson OC, Song Z, Chen H, et al. (2013) Fis1, Mff, MiD49, and MiD51 mediate Drp1 recruitment in mitochondrial fission. Mol Biol Cell 24: 659-667. https://doi.org/10.1091/mbc.E12-10-0721 ![]() |
[37] |
Lackner LL (2014) Shaping the dynamic mitochondrial network. BMC Biol 12: 35. https://doi.org/10.1186/1741-7007-12-35 ![]() |
[38] |
Denton RM (2009) Regulation of mitochondrial dehydrogenases by calcium ions. Biochim Biophys Acta 1787: 1309-1316. https://doi.org/10.1016/j.bbabio.2009.01.005 ![]() |
[39] |
Gellerich FN, Gizatullina Z, Trumbeckaite S, et al. (2010) The regulation of OXPHOS by extramitochondrial calcium. Biochim Biophys Acta 1797: 1018-1027. https://doi.org/10.1016/j.bbabio.2010.02.005 ![]() |
[40] |
Jana F, Bustos G, Rivas J, et al. (2019) Complex I and II are required for normal mitochondrial Ca2+ homeostasis. Mitochondrion 49: 73-82. https://doi.org/10.1016/j.mito.2019.07.004 ![]() |
[41] |
Balderas E, Eberhardt DR, Lee S, et al. (2022) Mitochondrial calcium uniporter stabilization preserves energetic homeostasis during complex I impairment. Nat Commun 13: 2769. https://doi.org/10.1038/s41467-022-30236-4 ![]() |
[42] |
Noyola-Martinez N, Halhali A, Barrera D (2019) Steroid hormones and pregnancy. Gynecol Endocrinol 35: 376-384. https://doi.org/10.1080/09513590.2018.1564742 ![]() |
[43] |
Russell JK, Jones CK, Newhouse PA (2019) The role of estrogen in brain and cognitive aging. Neurotherapeutics 16: 649-665. https://doi.org/10.1007/s13311-019-00766-9 ![]() |
[44] |
Soria-Jasso LE, Carino-Cortes R, Munoz-Perez VM, et al. (2019) Beneficial and deleterious effects of female sex hormones, oral contraceptives, and phytoestrogens by immunomodulation on the liver. Int J Mol Sci 20: 4694. https://doi.org/10.3390/ijms20194694 ![]() |
[45] |
Bernasochi GB, Bell JR, Simpson ER, et al. (2019) Impact of estrogens on the regulation of white, beige, and brown adipose tissue depots. Compr Physiol 9: 457-475. https://doi.org/10.1002/cphy.c180009 ![]() |
[46] |
Giatti S, Garcia-Segura LM, Barreto GE, et al. (2019) Neuroactive steroids, neurosteroidogenesis and sex. Prog Neurobiol 176: 1-17. https://doi.org/10.1016/j.pneurobio.2018.06.007 ![]() |
[47] |
Zhang H, Cui D, Wang B, et al. (2007) Pharmacokinetic drug interactions involving 17alpha-ethinylestradiol: A new look at an old drug. Clin Pharmacokinet 46: 133-157. https://doi.org/10.2165/00003088-200746020-00003 ![]() |
[48] |
Kuhl H (2005) Pharmacology of estrogens and progestogens: Influence of different routes of administration. Climacteric 8: 3-63. https://doi.org/10.1080/13697130500148875 ![]() |
[49] |
Kumar R, Zakharov MN, Khan SH, et al. (2011) The dynamic structure of the estrogen receptor. J Amino Acids 2011: 812540. https://doi.org/10.4061/2011/812540 ![]() |
[50] |
Gaudet HM, Cheng SB, Christensen EM, et al. (2015) The G-protein coupled estrogen receptor, GPER: The inside and inside-out story. Mol Cell Endocrinol 418: 207-219. https://doi.org/10.1016/j.mce.2015.07.016 ![]() |
[51] |
Yasar P, Ayaz G, User SD, et al. (2017) Molecular mechanism of estrogen-estrogen receptor signaling. Reprod Med Biol 16: 4-20. https://doi.org/10.1002/rmb2.12006 ![]() |
[52] |
Fuentes N, Silveyra P (2019) Estrogen receptor signaling mechanisms. Adv Protein Chem Struct Biol 116: 135-170. https://doi.org/10.1016/bs.apcsb.2019.01.001 ![]() |
[53] |
Li X, Zhang S, Safe S (2006) Activation of kinase pathways in MCF-7 cells by 17beta-estradiol and structurally diverse estrogenic compounds. J Steroid Biochem Mol Biol 98: 122-132. https://doi.org/10.1016/j.jsbmb.2005.08.018 ![]() |
[54] |
Alvarez-Delgado C (2022) The role of mitochondria and mitochondrial hormone receptors on the bioenergetic adaptations to lactation. Mol Cell Endocrinol 551: 111661. https://doi.org/10.1016/j.mce.2022.111661 ![]() |
[55] |
Chen JQ, Yager JD, Russo J (2005) Regulation of mitochondrial respiratory chain structure and function by estrogens/estrogen receptors and potential physiological/pathophysiological implications. Biochim Biophys Acta 1746: 1-17. https://doi.org/10.1016/j.bbamcr.2005.08.001 ![]() |
[56] |
Escande A, Pillon A, Servant N, et al. (2006) Evaluation of ligand selectivity using reporter cell lines stably expressing estrogen receptor alpha or beta. Biochem Pharmacol 71: 1459-1469. https://doi.org/10.1016/j.bcp.2006.02.002 ![]() |
[57] |
Jeyakumar M, Carlson KE, Gunther JR, et al. (2011) Exploration of dimensions of estrogen potency: Parsing ligand binding and coactivator binding affinities. J Biol Chem 286: 12971-12982. https://doi.org/10.1074/jbc.M110.205112 ![]() |
[58] |
Monje P, Boland R (2002) Expression and cellular localization of naturally occurring beta estrogen receptors in uterine and mammary cell lines. J Cell Biochem 86: 136-144. https://doi.org/10.1002/jcb.10193 ![]() |
[59] |
Chen J, Li Y, Lavigne JA, et al. (1999) Increased mitochondrial superoxide production in rat liver mitochondria, rat hepatocytes, and HepG2 cells following ethinyl estradiol treatment. Toxicol Sci 51: 224-235. https://doi.org/10.1093/toxsci/51.2.224 ![]() |
[60] |
Solakidi S, Psarra AMG, Sekeris CE (2005) Differential subcellular distribution of estrogen receptor isoforms: localization of ERalpha in the nucleoli and ERbeta in the mitochondria of human osteosarcoma SaOS-2 and hepatocarcinoma HepG2 cell lines. Biochim Biophys Acta 1745: 382-392. https://doi.org/10.1016/j.bbamcr.2005.05.010 ![]() |
[61] |
Cammarata PR, Chu S, Moor A, et al. (2004) Subcellular distribution of native estrogen receptor alpha and beta subtypes in cultured human lens epithelial cells. Exp Eye Res 78: 861-871. https://doi.org/10.1016/j.exer.2003.09.027 ![]() |
[62] |
Chen JQ, Delannoy M, Cooke C, et al. (2004) Mitochondrial localization of ERalpha and ERbeta in human MCF7 cells. Am J Physiol Endocrinol Metab 286: E1011-E1022. https://doi.org/10.1152/ajpendo.00508.2003 ![]() |
[63] |
Chen JQ, Eshete M, Alworth WL, et al. (2004) Binding of MCF-7 cell mitochondrial proteins and recombinant human estrogen receptors alpha and beta to human mitochondrial DNA estrogen response elements. J Cell Biochem 93: 358-373. https://doi.org/10.1002/jcb.20178 ![]() |
[64] |
Chen JQ, Yager JD (2004) Estrogen's effects on mitochondrial gene expression: mechanisms and potential contributions to estrogen carcinogenesis. Ann N Y Acad Sci 1028: 258-272. https://doi.org/10.1196/annals.1322.030 ![]() |
[65] |
Alvarez-Delgado C, Mendoza-Rodriguez CA, Picazo O, et al. (2010) Different expression of alpha and beta mitochondrial estrogen receptors in the aging rat brain: interaction with respiratory complex V. Exp Gerontol 45: 580-585. https://doi.org/10.1016/j.exger.2010.01.015 ![]() |
[66] |
Chen JQ, Cammarata PR, Baines CP, et al. (2009) Regulation of mitochondrial respiratory chain biogenesis by estrogens/estrogen receptors and physiological, pathological and pharmacological implications. Biochim Biophys Acta 1793: 1540-1570. https://doi.org/10.1016/j.bbamcr.2009.06.001 ![]() |
[67] |
Heine PA, Taylor JA, Iwamoto GA, et al. (2000) Increased adipose tissue in male and female estrogen receptor-alpha knockout mice. Proc Natl Acad Sci U S A 97: 12729-12734. https://doi.org/10.1073/pnas.97.23.12729 ![]() |
[68] |
Musatov S, Chen W, Pfaff DW, et al. (2007) Silencing of estrogen receptor alpha in the ventromedial nucleus of hypothalamus leads to metabolic syndrome. Proc Natl Acad Sci U S A 104: 2501-2506. https://doi.org/10.1073/pnas.0610787104 ![]() |
[69] |
Gao Q, Horvath TL (2008) Cross-talk between estrogen and leptin signaling in the hypothalamus. Am J Physiol Endocrinol Metab 294: E817-E826. https://doi.org/10.1152/ajpendo.00733.2007 ![]() |
[70] |
Foryst-Ludwig A, Clemenz M, Hohmann S, et al. (2008) Metabolic actions of estrogen receptor beta (ERbeta) are mediated by a negative cross-talk with PPARgamma. PLoS Genet 4: e1000108. https://doi.org/10.1371/journal.pgen.1000108 ![]() |
[71] |
Torres MJ, Kew KA, Ryan TE, et al. (2018) 17beta-Estradiol directly lowers mitochondrial membrane microviscosity and improves bioenergetic function in skeletal muscle. Cell Metab 27: 167-179. https://doi.org/10.1016/j.cmet.2017.10.003 ![]() |
[72] |
Torres MJ, Ryan TE, Lin CT, et al. (2018) Impact of 17beta-estradiol on complex I kinetics and H2O2 production in liver and skeletal muscle mitochondria. J Biol Chem 293: 16889-16898. https://doi.org/10.1074/jbc.RA118.005148 ![]() |
[73] |
Moreno AJM, Moreira PI, Custodio JBA, et al. (2013) Mechanism of inhibition of mitochondrial ATP synthase by 17beta-estradiol. J Bioenerg Biomembr 45: 261-270. https://doi.org/10.1007/s10863-012-9497-1 ![]() |
[74] |
Sastre J, Pallardo FV, Vina J (2003) The role of mitochondrial oxidative stress in aging. Free Radic Biol Med 35: 1-8. https://doi.org/10.1016/s0891-5849(03)00184-9 ![]() |
[75] |
Borras C, Gambini J, Vina J (2007) Mitochondrial oxidant generation is involved in determining why females live longer than males. Front Biosci 12: 1008-1013. https://doi.org/10.2741/2120 ![]() |
[76] |
Borras C, Gambini J, Gomez-Cabrera MC, et al. (2005) 17beta-oestradiol up-regulates longevity-related, antioxidant enzyme expression via the ERK1 and ERK2[MAPK]/NFkappaB cascade. Aging Cell 4: 113-118. https://doi.org/10.1111/j.1474-9726.2005.00151.x ![]() |
[77] |
Borras C, Gambini J, Lopez-Grueso R, et al. (2010) Direct antioxidant and protective effect of estradiol on isolated mitochondria. Biochim Biophys Acta 1802: 205-211. https://doi.org/10.1016/j.bbadis.2009.09.007 ![]() |
[78] |
La Colla A, Vasconsuelo A, Boland R (2013) Estradiol exerts antiapoptotic effects in skeletal myoblasts via mitochondrial PTP and MnSOD. J Endocrinol 216: 331-341. https://doi.org/10.1530/JOE-12-0486 ![]() |
[79] |
Bauza-Thorbrugge M, Galmés-Pascual BM, Sbert-Roig M, et al. (2017) Antioxidant peroxiredoxin 3 expression is regulated by 17beta-estradiol in rat white adipose tissue. J Steroid Biochem Mol Biol 172: 9-19. https://doi.org/10.1016/j.jsbmb.2017.05.008 ![]() |
[80] |
Kalkhoran SB, Kararigas G (2022) Oestrogenic regulation of mitochondrial dynamics. Int J Mol Sci 23: 1118. https://doi.org/10.3390/ijms23031118 ![]() |
[81] |
Tao Z, Cheng Z (2023) Hormonal regulation of metabolism-recent lessons learned from insulin and estrogen. Clin Sci (Lond) 137: 415-434. https://doi.org/10.1042/CS20210519 ![]() |
[82] |
Sastre-Serra J, Nadal-Serrano M, Pons DG, et al. (2012) Mitochondrial dynamics is affected by 17beta-estradiol in the MCF-7 breast cancer cell line. Effects on fusion and fission related genes. Int J Biochem Cell Biol 44: 1901-1905. https://doi.org/10.1016/j.biocel.2012.07.012 ![]() |
[83] |
Castracani CC, Longhitano L, Distefano A, et al. (2020) Role of 17beta-estradiol on cell proliferation and mitochondrial fitness in glioblastoma cells. J Oncol 2020: 2314693. https://doi.org/10.1155/2020/2314693 ![]() |
[84] |
Satohisa S, Zhang HH, Feng L, et al. (2014) Endogenous NO upon estradiol-17beta stimulation and NO donor differentially regulate mitochondrial S-nitrosylation in endothelial cells. Endocrinology 155: 3005-3016. https://doi.org/10.1210/en.2013-2174 ![]() |
[85] |
Zhou Z, Ribas V, Rajbhandari P, et al. (2018) Estrogen receptor α protects pancreatic beta-cells from apoptosis by preserving mitochondrial function and suppressing endoplasmic reticulum stress. J Biol Chem 293: 4735-4751. https://doi.org/10.1074/jbc.M117.805069 ![]() |
[86] |
Lobaton CD, Vay L, Hernandez-Sanmiguel E, et al. (2005) Modulation of mitochondrial Ca2+ uptake by estrogen receptor agonists and antagonists. Br J Pharmacol 145: 862-871. https://doi.org/10.1038/sj.bjp.0706265 ![]() |
[87] |
Vasan N, Toska E, Scaltriti M (2019) Overview of the relevance of PI3K pathway in HR-positive breast cancer. Ann Oncol 30: x3-x11. https://doi.org/10.1093/annonc/mdz281 ![]() |
[88] |
Marchi S, Corricelli M, Branchini A, et al. (2019) Akt-mediated phosphorylation of MICU1 regulates mitochondrial Ca2+ levels and tumor growth. EMBO J 38: e99435. https://doi.org/10.15252/embj.201899435 ![]() |
[89] |
Qin S, Yin J, Huang K (2016) Free fatty acids increase intracellular lipid accumulation and oxidative stress by modulating PPARalpha and SREBP-1c in L-02 cells. Lipids 51: 797-805. https://doi.org/10.1007/s11745-016-4160-y ![]() |
[90] |
Colak E, Pap D (2021) The role of oxidative stress in the development of obesity and obesity-related metabolic disorders. J Med Biochem 40: 1-9. https://doi.org/10.5937/jomb0-24652 ![]() |
[91] |
Petersen MC, Shulman GI (2018) Mechanisms of insulin action and insulin resistance. Physiol Rev 98: 2133-2223. https://doi.org/10.1152/physrev.00063.2017 ![]() |
[92] |
Kruger M, Kratchmarova I, Blagoev B, et al. (2008) Dissection of the insulin signaling pathway via quantitative phosphoproteomics. Proc Natl Acad Sci U S A 105: 2451-2456. https://doi.org/10.1073/pnas.0711713105 ![]() |
[93] |
Taniguchi CM, Emanuelli B, Kahn CR (2006) Critical nodes in signalling pathways: insights into insulin action. Nat Rev Mol Cell Biol 7: 85-96. https://doi.org/10.1038/nrm1837 ![]() |
[94] |
Boura-Halfon S, Zick Y (2009) Phosphorylation of IRS proteins, insulin action, and insulin resistance. Am J Physiol Endocrinol Metab 296: E581-E591. https://doi.org/10.1152/ajpendo.90437.2008 ![]() |
[95] |
Petersen KF, Befroy D, Dufour S, et al. (2003) Mitochondrial dysfunction in the elderly: Possible role in insulin resistance. Science 300: 1140-1142. https://doi.org/10.1126/science.1082889 ![]() |
[96] |
Kelley DE, Goodpaster B, Wing RR, et al. (1999) Skeletal muscle fatty acid metabolism in association with insulin resistance, obesity, and weight loss. Am J Physiol 277: E1130-E1141. https://doi.org/10.1152/ajpendo.1999.277.6.E1130 ![]() |
[97] |
Simoneau JA, Veerkamp JH, Turcotte LP, et al. (1999) Markers of capacity to utilize fatty acids in human skeletal muscle: relation to insulin resistance and obesity and effects of weight loss. FASEB J 13: 2051-2060. https://doi.org/10.1096/fasebj.13.14.2051 ![]() |
[98] |
Kim JY, Hickner RC, Cortright RL, et al. (2000) Lipid oxidation is reduced in obese human skeletal muscle. Am J Physiol Endocrinol Metab 279: E1039-E1044. https://doi.org/10.1152/ajpendo.2000.279.5.E1039 ![]() |
[99] |
Szendroedi J, Phielix E, Roden M (2011) The role of mitochondria in insulin resistance and type 2 diabetes mellitus. Nat Rev Endocrinol 8: 92-103. https://doi.org/10.1038/nrendo.2011.138 ![]() |
[100] |
San-Millan I (2023) The key role of mitochondrial function in health and disease. Antioxidants (Basel) 12: 782. https://doi.org/10.3390/antiox12040782 ![]() |
[101] |
Morino K, Petersen KF, Dufour S, et al. (2005) Reduced mitochondrial density and increased IRS-1 serine phosphorylation in muscle of insulin-resistant offspring of type 2 diabetic parents. J Clin Invest 115: 3587-3593. https://doi.org/10.1172/JCI25151 ![]() |
[102] |
Ritov VB, Menshikova EV, He J, et al. (2005) Deficiency of subsarcolemmal mitochondria in obesity and type 2 diabetes. Diabetes 54: 8-14. https://doi.org/10.2337/diabetes.54.1.8 ![]() |
[103] |
Chomentowski P, Coen PM, Radikova Z, et al. (2011) Skeletal muscle mitochondria in insulin resistance: differences in intermyofibrillar versus subsarcolemmal subpopulations and relationship to metabolic flexibility. J Clin Endocrinol Metab 96: 494-503. https://doi.org/10.1210/jc.2010-0822 ![]() |
[104] |
Amati F, Dube JJ, Alvarez-Carnero E, et al. (2011) Skeletal muscle triglycerides, diacylglycerols, and ceramides in insulin resistance: Another paradox in endurance-trained athletes?. Diabetes 60: 2588-2597. https://doi.org/10.2337/db10-1221 ![]() |
[105] |
Bergman BC, Goodpaster BH (2020) Exercise and muscle lipid content, composition, and localization: Influence on muscle insulin sensitivity. Diabetes 69: 848-858. https://doi.org/10.2337/dbi18-0042 ![]() |
[106] |
Sergi D, Naumovski N, Heilbronn LK, et al. (2019) Mitochondrial (dys)function and insulin resistance: From pathophysiological molecular mechanisms to the impact of diet. Front Physiol 10: 532. https://doi.org/10.3389/fphys.2019.00532 ![]() |
[107] |
Holloszy JO (2009) Skeletal muscle “mitochondrial deficiency” does not mediate insulin resistance. Am J Clin Nutr 89: 463S-466S. https://doi.org/10.3945/ajcn.2008.26717C ![]() |
[108] |
Asmann YW, Stump CS, Short KR, et al. (2006) Skeletal muscle mitochondrial functions, mitochondrial DNA copy numbers, and gene transcript profiles in type 2 diabetic and nondiabetic subjects at equal levels of low or high insulin and euglycemia. Diabetes 55: 3309-3319. https://doi.org/10.2337/db05-1230 ![]() |
[109] |
Mogensen M, Sahlin K, Fernstrom M, et al. (2007) Mitochondrial respiration is decreased in skeletal muscle of patients with type 2 diabetes. Diabetes 56: 1592-1599. https://doi.org/10.2337/db06-0981 ![]() |
[110] |
Phielix E, Schrauwen-Hinderling VB, Mensink M, et al. (2008) Lower intrinsic ADP-stimulated mitochondrial respiration underlies in vivo mitochondrial dysfunction in muscle of male type 2 diabetic patients. Diabetes 57: 2943-2949. https://doi.org/10.2337/db08-0391 ![]() |
[111] |
Koska J, Stefan N, Permana PA, et al. (2008) Increased fat accumulation in liver may link insulin resistance with subcutaneous abdominal adipocyte enlargement, visceral adiposity, and hypoadiponectinemia in obese individuals. Am J Clin Nutr 87: 295-302. https://doi.org/10.1093/ajcn/87.2.295 ![]() |
[112] |
Petersen KF, Dufour S, Befroy D, et al. (2005) Reversal of nonalcoholic hepatic steatosis, hepatic insulin resistance, and hyperglycemia by moderate weight reduction in patients with type 2 diabetes. Diabetes 54: 603-608. https://doi.org/10.2337/diabetes.54.3.603 ![]() |
[113] |
Zhang D, Liu ZX, Choi CS, et al. (2007) Mitochondrial dysfunction due to long-chain Acyl-CoA dehydrogenase deficiency causes hepatic steatosis and hepatic insulin resistance. Proc Natl Acad Sci U S A 104: 17075-17080. https://doi.org/10.1073/pnas.0707060104 ![]() |
[114] |
Zhang D, Christianson J, Liu ZX, et al. (2010) Resistance to high-fat diet-induced obesity and insulin resistance in mice with very long-chain acyl-CoA dehydrogenase deficiency. Cell Metab 11: 402-411. https://doi.org/10.1016/j.cmet.2010.03.012 ![]() |
[115] |
Koliaki C, Szendroedi J, Kaul K, et al. (2015) Adaptation of hepatic mitochondrial function in humans with non-alcoholic fatty liver is lost in steatohepatitis. Cell Metab 21: 739-746. https://doi.org/10.1016/j.cmet.2015.04.004 ![]() |
[116] |
Schmid AI, Szendroedi J, Chmelik M, et al. (2011) Liver ATP synthesis is lower and relates to insulin sensitivity in patients with type 2 diabetes. Diabetes Care 34: 448-453. https://doi.org/10.2337/dc10-1076 ![]() |
[117] |
Gancheva S, Kahl S, Pesta D, et al. (2022) Impaired hepatic mitochondrial capacity in nonalcoholic steatohepatitis associated with type 2 diabetes. Diabetes Care 45: 928-937. https://doi.org/10.2337/dc21-1758 ![]() |
[118] |
De Pauw A, Tejerina S, Raes M, et al. (2009) Mitochondrial (dys)function in adipocyte (de)differentiation and systemic metabolic alterations. Am J Pathol 175: 927-939. https://doi.org/10.2353/ajpath.2009.081155 ![]() |
[119] |
Lee JH, Park A, Oh KJ, et al. (2019) The role of adipose tissue mitochondria: Regulation of mitochondrial function for the treatment of metabolic diseases. Int J Mol Sci 20: 4924. https://doi.org/10.3390/ijms20194924 ![]() |
[120] |
Chattopadhyay M, Guhathakurta I, Behera P, et al. (2011) Mitochondrial bioenergetics is not impaired in nonobese subjects with type 2 diabetes mellitus. Metabolism 60: 1702-1710. https://doi.org/10.1016/j.metabol.2011.04.015 ![]() |
[121] |
Heinonen S, Buzkova J, Muniandy M, et al. (2015) Impaired mitochondrial biogenesis in adipose tissue in acquired obesity. Diabetes 64: 3135-3145. https://doi.org/10.2337/db14-1937 ![]() |
[122] |
Bohm A, Keuper M, Meile T, et al. (2020) Increased mitochondrial respiration of adipocytes from metabolically unhealthy obese compared to healthy obese individuals. Sci Rep 10: 12407. https://doi.org/10.1038/s41598-020-69016-9 ![]() |
[123] |
Meister BM, Hong SG, Shin J, et al. (2022) Healthy versus unhealthy adipose tissue expansion: The role of exercise. J Obes Metab Syndr 31: 37-50. https://doi.org/10.7570/jomes21096 ![]() |
[124] |
Choo HJ, Kim JH, Kwon OB, et al. (2006) Mitochondria are impaired in the adipocytes of type 2 diabetic mice. Diabetologia 49: 784-791. https://doi.org/10.1007/s00125-006-0170-2 ![]() |
[125] |
Rong JX, Qiu Y, Hansen MK, et al. (2007) Adipose mitochondrial biogenesis is suppressed in db/db and high-fat diet-fed mice and improved by rosiglitazone. Diabetes 56: 1751-1760. https://doi.org/10.2337/db06-1135 ![]() |
[126] |
Komatsu M, Takei M, Ishii H, et al. (2013) Glucose-stimulated insulin secretion: A newer perspective. J Diabetes Investig 4: 511-516. https://doi.org/10.1111/jdi.12094 ![]() |
[127] |
Anello M, Lupi R, Spampinato D, et al. (2005) Functional and morphological alterations of mitochondria in pancreatic beta cells from type 2 diabetic patients. Diabetologia 48: 282-289. https://doi.org/10.1007/s00125-004-1627-9 ![]() |
[128] |
Saxena R, de Bakker PI, Singer K, et al. (2006) Comprehensive association testing of common mitochondrial DNA variation in metabolic disease. Am J Hum Genet 79: 54-61. https://doi.org/10.1086/504926 ![]() |
[129] |
Koeck T, Olsson AH, Nitert MD, et al. (2011) A common variant in TFB1M is associated with reduced insulin secretion and increased future risk of type 2 diabetes. Cell Metab 13: 80-91. https://doi.org/10.1016/j.cmet.2010.12.007 ![]() |
[130] |
Sharoyko VV, Abels M, Sun J, et al. (2014) Loss of TFB1M results in mitochondrial dysfunction that leads to impaired insulin secretion and diabetes. Hum Mol Genet 23: 5733-5749. https://doi.org/10.1093/hmg/ddu288 ![]() |
[131] |
Sidarala V, Pearson GL, Parekh VS, et al. (2020) Mitophagy protects β cells from inflammatory damage in diabetes. JCI Insight 5: e141138. https://doi.org/10.1172/jci.insight.141138 ![]() |
[132] |
Vezza T, Diaz-Pozo P, Canet F, et al. (2022) The role of mitochondrial dynamic dysfunction in age-associated type 2 diabetes. World J Mens Health 40: 399-411. https://doi.org/10.5534/wjmh.210146 ![]() |
[133] |
Shenouda SM, Widlansky ME, Chen K, et al. (2011) Altered mitochondrial dynamics contributes to endothelial dysfunction in diabetes mellitus. Circulation 124: 444-453. https://doi.org/10.1161/CIRCULATIONAHA.110.014506 ![]() |
[134] |
Hu Q, Zhang H, Cortes NG, et al. (2020) Increased Drp1 acetylation by lipid overload induces cardiomyocyte death and heart dysfunction. Circ Res 126: 456-470. https://doi.org/10.1161/CIRCRESAHA.119.315252 ![]() |
[135] |
Sidarala V, Zhu J, Levi-D'Ancona E, et al. (2022) Mitofusin 1 and 2 regulation of mitochondrial DNA content is a critical determinant of glucose homeostasis. Nat Commun 13: 2340. https://doi.org/10.1038/s41467-022-29945-7 ![]() |
[136] |
Tubbs E, Chanon S, Robert M, et al. (2018) Disruption of mitochondria-associated endoplasmic reticulum membrane (MAM) integrity contributes to muscle insulin resistance in mice and humans. Diabetes 67: 636-650. https://doi.org/10.2337/db17-0316 ![]() |
[137] |
Nieblas B, Perez-Trevino P, Garcia N (2022) Role of mitochondria-associated endoplasmic reticulum membranes in insulin sensitivity, energy metabolism, and contraction of skeletal muscle. Front Mol Biosci 9: 959844. https://doi.org/10.3389/fmolb.2022.959844 ![]() |
[138] |
Dror V, Kalynyak TB, Bychkivska Y, et al. (2008) Glucose and endoplasmic reticulum calcium channels regulate HIF-1beta via presenilin in pancreatic beta-cells. J Biol Chem 283: 9909-9916. https://doi.org/10.1074/jbc.M710601200 ![]() |
[139] |
Koval OM, Nguyen EK, Mittauer DJ, et al. (2023) Regulation of smooth muscle cell proliferation by mitochondrial Ca2+ in type 2 Diabetes. Int J Mol Sci 24: 12897. https://doi.org/10.3390/ijms241612897 ![]() |
[140] |
Janssen I, Powell LH, Crawford S, et al. (2008) Menopause and the metabolic syndrome: The study of women's health across the nation. Arch Intern Med 168: 1568-1575. https://doi.org/10.1001/archinte.168.14.1568 ![]() |
[141] |
Oya J, Nakagami T, Yamamoto Y, et al. (2014) Effects of age on insulin resistance and secretion in subjects without diabetes. Int Med 53: 941-947. https://doi.org/10.2169/internalmedicine.53.1580 ![]() |
[142] |
Pu D, Tan R, Yu Q, et al. (2017) Metabolic syndrome in menopause and associated factors: A meta-analysis. Climacteric 20: 583-591. https://doi.org/10.1080/13697137.2017.1386649 ![]() |
[143] |
Korljan B, Bagatin J, Kokic S, et al. (2010) The impact of hormone replacement therapy on metabolic syndrome components in perimenopausal women. Med Hypotheses 74: 162-163. https://doi.org/10.1016/j.mehy.2009.07.008 ![]() |
[144] |
Lobo RA (2017) Hormone-replacement therapy: Current thinking. Nat Rev Endocrinol 13: 220-231. https://doi.org/10.1038/nrendo.2016.164 ![]() |
[145] |
Bitoska I, Krstevska B, Milenkovic T, et al. (2016) Effects of hormone replacement therapy on insulin resistance in postmenopausal diabetic women. Open Access Maced J Med Sci 4: 83-88. https://doi.org/10.3889/oamjms.2016.024 ![]() |
[146] |
Weigt C, Hertrampf T, Flenker U, et al. (2015) Effects of estradiol, estrogen receptor subtype-selective agonists and genistein on glucose metabolism in leptin resistant female Zucker diabetic fatty (ZDF) rats. J Steroid Biochem Mol Biol 154: 12-22. https://doi.org/10.1016/j.jsbmb.2015.06.002 ![]() |
[147] |
Ribas V, Drew BG, Zhou Z, et al. (2016) Skeletal muscle action of estrogen receptor alpha is critical for the maintenance of mitochondrial function and metabolic homeostasis in females. Sci Transl Med 8: 334ra354. https://doi.org/10.1126/scitranslmed.aad3815 ![]() |
[148] |
Diaz A, Lopez-Grueso R, Gambini J, et al. (2019) Sex differences in age-associated type 2 diabetes in rats-role of estrogens and oxidative stress. Oxid Med Cell Longev 2019: 6734836. https://doi.org/10.1155/2019/6734836 ![]() |
[149] |
Le May C, Chu K, Hu M, et al. (2006) Estrogens protect pancreatic beta-cells from apoptosis and prevent insulin-deficient diabetes mellitus in mice. Proc Natl Acad Sci U S A 103: 9232-9237. https://doi.org/10.1073/pnas.0602956103 ![]() |
[150] |
Alonso-Magdalena P, Ropero AB, Carrera MP, et al. (2008) Pancreatic insulin content regulation by the estrogen receptor ER alpha. PLoS One 3: e2069. https://doi.org/10.1371/journal.pone.0002069 ![]() |
[151] |
Kilic G, Alvarez-Mercado AI, Zarrouki B, et al. (2014) The islet estrogen receptor-α is induced by hyperglycemia and protects against oxidative stress-induced insulin-deficient diabetes. PLoS One 9: e87941. https://doi.org/10.1371/journal.pone.0087941 ![]() |
[152] |
Liu S, Le May C, Wong WPS, et al. (2009) Importance of extranuclear estrogen receptor-alpha and membrane G protein-coupled estrogen receptor in pancreatic islet survival. Diabetes 58: 2292-2302. https://doi.org/10.2337/db09-0257 ![]() |
[153] |
Hevener A, Reichart D, Janez A, et al. (2002) Female rats do not exhibit free fatty acid-induced insulin resistance. Diabetes 51: 1907-1912. https://doi.org/10.2337/diabetes.51.6.1907 ![]() |
[154] |
Camporez JP, Lyu K, Goldberg EL, et al. (2019) Anti-inflammatory effects of oestrogen mediate the sexual dimorphic response to lipid-induced insulin resistance. J Physiol 597: 3885-3903. https://doi.org/10.1113/JP277270 ![]() |
[155] |
Gonzalez-Granillo M, Savva C, Li X, et al. (2019) ERβ activation in obesity improves whole body metabolism via adipose tissue function and enhanced mitochondria biogenesis. Mol Cell Endocrinol 479: 147-158. https://doi.org/10.1016/j.mce.2018.10.007 ![]() |
[156] |
Galmes-Pascual BM, Martinez-Cignoni MR, Moran-Costoya A, et al. (2020) 17β-estradiol ameliorates lipotoxicity-induced hepatic mitochondrial oxidative stress and insulin resistance. Free Radic Biol Med 150: 148-160. https://doi.org/10.1016/j.freeradbiomed.2020.02.016 ![]() |
[157] |
Nauck MA, Vardarli I, Deacon CF, et al. (2011) Secretion of glucagon-like peptide-1 (GLP-1) in type 2 diabetes: What is up, what is down?. Diabetologia 54: 10-18. https://doi.org/10.1007/s00125-010-1896-4 ![]() |
[158] |
Drucker DJ, Nauck MA (2006) The incretin system: Glucagon-like peptide-1 receptor agonists and dipeptidyl peptidase-4 inhibitors in type 2 diabetes. Lancet 368: 1696-1705. https://doi.org/10.1016/S0140-6736(06)69705-5 ![]() |
[159] |
Buteau J (2008) GLP-1 receptor signaling: effects on pancreatic beta-cell proliferation and survival. Diabetes Metab 34: S73-S77. https://doi.org/10.1016/S1262-3636(08)73398-6 ![]() |
[160] |
Tiano JP, Tate CR, Yang BS, et al. (2015) Effect of targeted estrogen delivery using glucagon-like peptide-1 on insulin secretion, insulin sensitivity and glucose homeostasis. Sci Rep 5: 10211. https://doi.org/10.1038/srep10211 ![]() |
[161] |
Fuselier T, de Sa PM, Qadir MMF, et al. (2022) Efficacy of glucagon-like peptide-1 and estrogen dual agonist in pancreatic islets protection and pre-clinical models of insulin-deficient diabetes. Cell Rep Med 3: 100598. https://doi.org/10.1016/j.xcrm.2022.100598 ![]() |
[162] |
Finan B, Yang B, Ottaway N, et al. (2012) Targeted estrogen delivery reverses the metabolic syndrome. Nat Med 18: 1847-1856. https://doi.org/10.1038/nm.3009 ![]() |
[163] | Jiang Q, Yin J, Chen J, et al. (2020) Mitochondria-targeted antioxidants: A step towards disease treatment. Oxid Med Cell Longev 2020: 8837893. https://doi.org/10.1155/2020/8837893 |
[164] |
Zielonka J, Joseph J, Sikora A, et al. (2017) Mitochondria-targeted triphenylphosphonium-based compounds: Syntheses, mechanisms of action, and therapeutic and diagnostic applications. Chem Rev 117: 10043-10120. https://doi.org/10.1021/acs.chemrev.7b00042 ![]() |
[165] |
Cheng G, Zielonka J, McAllister DM, et al. (2013) Mitochondria-targeted vitamin E analogs inhibit breast cancer cell energy metabolism and promote cell death. BMC Cancer 13: 285. https://doi.org/10.1186/1471-2407-13-285 ![]() |
[166] |
Kelso GF, Porteous CM, Coulter CV, et al. (2001) Selective targeting of a redox-active ubiquinone to mitochondria within cells: antioxidant and antiapoptotic properties. J Biol Chem 276: 4588-4596. https://doi.org/10.1074/jbc.M009093200 ![]() |
1. | Dennis Opoku Boadu, Justice Kwame Appati, Joseph Agyapong Mensah, Exploring the Effectiveness of Graph-based Computational Models in COVID-19 Research, 2024, 5, 2662-2556, 10.1007/s43069-024-00362-4 |
Users | Time | Points |
10 | 0.29 | 837 |
20 | 0.41 | 1755 |
30 | 0.72 | 2565 |
40 | 0.94 | 3595 |
50 | 1.20 | 4954 |
100 | 3.60 | 10746 |
150 | 7.68 | 15397 |