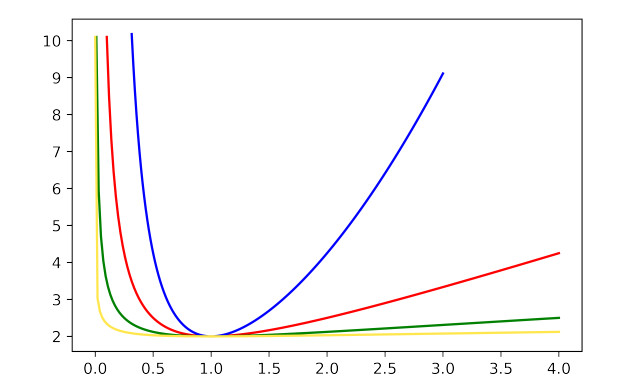
Carbon-based nanostructured materials are very promising for spintronic applications due to their weak spin-orbit coupling and potentially providing a long spin lifetime. Nanostructured carbons are not magnetic materials, but intrinsic magnetic behavioral nanostructure carbon materials could be fabricated through qualitative alterations. On alterations of carbon nanostructured materials, it changes their critical temperature and magneto-crystalline anisotropy energy that could be useful as favorable magnetic materials for different magnetic/electromagnetic device-based applications. Different processes are used for the alteration of nanostructure carbon materials like chemical doping, introducing defects, changing the density of states, functionalization, intercalation, forming heterostructure and fabricating nanocomposites layered semiconductor materials. Among the carbon-based derived nanostructured materials, the graphene oxide (GO) gets attracted towards the magnet forming in the spin-like structure across the area of the magnet. Due to its magnetic behaviour, it is used for the adsorption of metals and radionuclides and to make nonconductive oxide-metal. In this review article, the basics of magnetic behavioral change of the carbon-based GO/GO-nanocomposites nanostructured materials are described by gathering information from the literature that were/are reported by different researchers/research groups worldwide.
Citation: Sekhar Chandra Ray. Possible magnetic performances of graphene-oxide and it's composites: A brief review[J]. AIMS Materials Science, 2023, 10(5): 767-818. doi: 10.3934/matersci.2023043
[1] | Jingli Xie, Hongli Guo, Meiyang Zhang . Dynamics of an SEIR model with media coverage mediated nonlinear infectious force. Mathematical Biosciences and Engineering, 2023, 20(8): 14616-14633. doi: 10.3934/mbe.2023654 |
[2] | Martin Luther Mann Manyombe, Joseph Mbang, Jean Lubuma, Berge Tsanou . Global dynamics of a vaccination model for infectious diseases with asymptomatic carriers. Mathematical Biosciences and Engineering, 2016, 13(4): 813-840. doi: 10.3934/mbe.2016019 |
[3] | Hamdy M. Youssef, Najat A. Alghamdi, Magdy A. Ezzat, Alaa A. El-Bary, Ahmed M. Shawky . A new dynamical modeling SEIR with global analysis applied to the real data of spreading COVID-19 in Saudi Arabia. Mathematical Biosciences and Engineering, 2020, 17(6): 7018-7044. doi: 10.3934/mbe.2020362 |
[4] | Abdisa Shiferaw Melese, Oluwole Daniel Makinde, Legesse Lemecha Obsu . Mathematical modelling and analysis of coffee berry disease dynamics on a coffee farm. Mathematical Biosciences and Engineering, 2022, 19(7): 7349-7373. doi: 10.3934/mbe.2022347 |
[5] | Yujie Sheng, Jing-An Cui, Songbai Guo . The modeling and analysis of the COVID-19 pandemic with vaccination and isolation: a case study of Italy. Mathematical Biosciences and Engineering, 2023, 20(3): 5966-5992. doi: 10.3934/mbe.2023258 |
[6] | María Guadalupe Vázquez-Peña, Cruz Vargas-De-León, Jorge Fernando Camacho-Pérez, Jorge Velázquez-Castro . Analysis and Bayesian estimation of a model for Chikungunya dynamics with relapse: An outbreak in Acapulco, Mexico. Mathematical Biosciences and Engineering, 2023, 20(10): 18123-18145. doi: 10.3934/mbe.2023805 |
[7] | S. J. Gutowska, K. A. Hoffman, K. F. Gurski . The effect of PrEP uptake and adherence on the spread of HIV in the presence of casual and long-term partnerships. Mathematical Biosciences and Engineering, 2022, 19(12): 11903-11934. doi: 10.3934/mbe.2022555 |
[8] | Maryam Al-Yahyai, Fatma Al-Musalhi, Ibrahim Elmojtaba, Nasser Al-Salti . Mathematical analysis of a COVID-19 model with different types of quarantine and isolation. Mathematical Biosciences and Engineering, 2023, 20(1): 1344-1375. doi: 10.3934/mbe.2023061 |
[9] | Yiwei He, Qianqian Cui, Zengyun Hu . Modeling and analysis of the transmission dynamics of cystic echinococcosis: Effects of increasing the number of sheep. Mathematical Biosciences and Engineering, 2023, 20(8): 14596-14615. doi: 10.3934/mbe.2023653 |
[10] | Rajanish Kumar Rai, Pankaj Kumar Tiwari, Yun Kang, Arvind Kumar Misra . Modeling the effect of literacy and social media advertisements on the dynamics of infectious diseases. Mathematical Biosciences and Engineering, 2020, 17(5): 5812-5848. doi: 10.3934/mbe.2020311 |
Carbon-based nanostructured materials are very promising for spintronic applications due to their weak spin-orbit coupling and potentially providing a long spin lifetime. Nanostructured carbons are not magnetic materials, but intrinsic magnetic behavioral nanostructure carbon materials could be fabricated through qualitative alterations. On alterations of carbon nanostructured materials, it changes their critical temperature and magneto-crystalline anisotropy energy that could be useful as favorable magnetic materials for different magnetic/electromagnetic device-based applications. Different processes are used for the alteration of nanostructure carbon materials like chemical doping, introducing defects, changing the density of states, functionalization, intercalation, forming heterostructure and fabricating nanocomposites layered semiconductor materials. Among the carbon-based derived nanostructured materials, the graphene oxide (GO) gets attracted towards the magnet forming in the spin-like structure across the area of the magnet. Due to its magnetic behaviour, it is used for the adsorption of metals and radionuclides and to make nonconductive oxide-metal. In this review article, the basics of magnetic behavioral change of the carbon-based GO/GO-nanocomposites nanostructured materials are described by gathering information from the literature that were/are reported by different researchers/research groups worldwide.
By a graph G=(V(G),E(G)), with V(G) and E(G) the set of vertices and edges of G respectively, we mean an undirected simple graph without isolated vertices (i.e., each vertex has at least a neighbor).
Given a graph G, representing a chemical structure,
X(G)=∑uv∈E(G)F(du,dv) |
is said a topological descriptor and, if also it correlates with a molecular property, it is called a topological index. Above, by uv we mean the edge of a graph G joining the vertices u and v, du denotes the degree of u, and F is an appropriate chosen function. Remarkably, topological indices capture physical properties of a chemical compound in a single number.
A great number of topological indices have been defined and studied over more than four decades. Among them, probably the most popular topological indices are the Randić and the Zagreb indices. The first and second Zagreb indices, denoted by M1 and M2, respectively, were defined by Gutman and Trinajstić (see [1]) in 1972 by
M1(G)=∑u∈V(G)d2u,M2(G)=∑uv∈E(G)dudv. |
For more details of the applications and mathematical properties of Zagreb indices see [2,3,4], and the references therein. Zagreb indices have many connections with other topological indices, see e.g., [5,6].
The concept of variable molecular descriptors was proposed as a way of characterizing heteroatoms (see [7,8]), but also to assess structural differences in alkylcycloalkanes [9]. The idea behind the variable molecular descriptors is that the variables are determined during the regression in order to minimize the error of estimate for a particular chemical property (see, e.g., [10]).
In this line of ideas, the variable versions of the first and second Zagreb indices were introduced as [10,11,12]
Mα1(G)=∑u∈V(G)dαu,Mα2(G)=∑uv∈E(G)(dudv)α, |
with α∈R. Evidently, M21 and M12 are the first and second Zagreb indices, respectively. In addition, the first and second variable Zagreb indices include several known indices. As examples we note that M−1/22 is the Randić index, M31 is the forgotten index F, M−11 is the inverse index ID, and M−12 is the modified Zagreb index.
In 2011, Vukičević proposed the variable symmetric division deg index [13]
SDDα(G)=∑uv∈E(G)(dαudαv+dαvdαu). | (1.1) |
Note that SDD−α(G)=SDDα(G) and so, it suffices to consider positive values of α. The symmetric division deg index is the best predictor of total surface area for polychlorobiphenyls [14].
In this work we perform studies of the variable symmetric division deg index from analytical and computational viewpoints. We obtain new inequalities for the variable symmetric division deg index SDDα(G) and we characterize graphs extremal with respect to them. Some of these inequalities generalize and improve previous results for the symmetric division deg index. In addition, we computationally apply the SDDα(G) index on random graphs and we demonstrate that the ratio ⟨SDDα(G)⟩/n (n denotes the order of the graph) depends only on the average degree ⟨d⟩.
One of our main results is Theorem 8, which provides upper and lower bounds of SDDα(G) in terms of the number of edges, the maximum and the minimum degree of G.
Let us start by proving a monotonicity property of these indices.
Theorem 1. Let G be a graph and 0<α<β.Then
SDDα(G)≤SDDβ(G), |
and the equality in the bound is attained if and only if each connected component of G is a regular graph.
Proof. Let us consider x≥1. Thus, xα≥x−β and
xβ−α−1≥0,xα(xβ−α−1)≥x−β(xβ−α−1),xβ−xα≥x−α−x−β,xβ+x−β≥xα+x−α, |
for every x≥1. Since u(x)=xα+x−α satisfies u(1/x)=u(x) for every x>0, we have xβ+x−β≥xα+x−α for every x>0. Note that we obtain the equality if and only if x=1.
Thus, we have
SDDβ(G)=∑uv∈E(G)(dβudβv+dβvdβu)≥∑uv∈E(G)(dαudαv+dαvdαu)=SDDα(G). |
The previous argument gives that we have the equality in the bound if and only if du/dv=1 for every uv∈E(G), i.e., each connected component of G is a regular graph.
Our next results in this section provide bounds of SDDα(G) involving the maximum and minimum degree of the graph G. Since scientists often estimate average degree of large networks, we present in the next section results involving the average degree.
Our next theorem relates the SDDα and the variable Zagreb indices.
Theorem 2. If G is a graph with minimum degree δ and maximum degree Δ, and α>0, then
2δ2αM−α2(G)≤SDDα(G)≤2Δ2αM−α2(G),Δ−2αM2α+11(G)≤SDDα(G)≤δ−2αM2α+11(G), |
and we have the equality in each bound if and only if G is regular.
Proof. First of all recall that for every function f the following equality
∑uv∈E(G)(f(du)+f(dv))=∑u∈V(G)duf(du) |
holds. In particular,
∑uv∈E(G)(d2αu+d2αv)=∑u∈V(G)d2α+1u=M2a+11(G). |
Since
SDDα(G)=∑uv∈E(G)(dαudαv+dαvdαu)=∑uv∈E(G)d2αu+d2αv(dudv)α. |
and α>0, we obtain
SDDα(G)=∑uv∈E(G)d2αu+d2αv(dudv)α≤2Δ2α∑uv∈E(G)(dudv)−α=2Δ2αM−α2(G), |
and
SDDα(G)=∑uv∈E(G)d2αu+d2αv(dudv)α≥2δ2α∑uv∈E(G)(dudv)−α=2δ2αM−α2(G). |
We also have
SDDα(G)=∑uv∈E(G)d2αu+d2αv(dudv)α≤δ−2α∑uv∈E(G)(d2αu+d2αv)=δ−2α∑u∈V(G)d2α+1u=δ−2αM2α+11(G), |
and
SDDα(G)=∑uv∈E(G)d2αu+d2αv(dudv)α≥Δ−2α∑uv∈E(G)(d2αu+d2αv)=Δ−2α∑u∈V(G)d2α+1u=Δ−2αM2α+11(G). |
If G is a regular graph, then each lower bound and its corresponding upper bound are the same, and both are equal to SDDα(G).
Assume now that the equality in either the first or second bound holds. The previous argument gives that we have either d2αu+d2αv=2Δ2α for any uv∈E(G) or d2αu+d2αv=2δ2α for any uv∈E(G). Since α>0, we have δ2α≤d2αu,d2αv≤Δ2α, and we conclude that du=dv=Δ for any uv∈E(G), or du=dv=δ for any uv∈E(G). Hence, G is a regular graph.
Finally, assume that the equality in either the third or fourth bound holds. The previous argument gives that we have either (dudv)α=δ2α for any uv∈E(G) or (dudv)α=Δ2α for any uv∈E(G). Since α>0, we have δα≤dαu,dαv≤Δα, and we conclude that du=dv=δ for any uv∈E(G), or du=dv=Δ for every uv∈E(G). Therefore, G is a regular graph.
We will need the following technical result.
Lemma 3. Let 0<a<A. Then
a≤x2+y2x+y≤A |
for every a≤x,y≤A.The lower bound is attained if and only if x=y=a.The upper bound is attained if and only if x=y=A.
Proof. If a≤x,y≤A, then ax+ay≤x2+y2≤Ax+Ay, and the statement holds.
A large kind of topological indices, named Adriatic indices, was introduced in [14,15]. Twenty of them were selected as significant predictors of chemical properties. One of them, the inverse sum indeg index, defined by
ISI(G)=∑uv∈E(G)dudvdu+dv=∑uv∈E(G)11du+1dv. |
appears in [14,15] as a good predictor of total surface area of octane isomers.
Next, we relate SDDα(G) with the variable inverse sum deg index defined, for each a∈R, as
ISDa(G)=∑uv∈E(G)1dau+dav. |
Note that ISD−1 is the inverse sum indeg index ISI.
Theorem 4. If G is a graph with m edges and minimum degree δ, and α>0, then
SDDα(G)≥δαm2ISD−α(G), |
and the equality in the bound holds if and only if G is regular.
Proof. Lemma 3 gives
δα≤x2α+y2αxα+yα≤Δα,1x2α+y2α≤δ−αxα+yα,1d2αu+d2αv≤δ−αdαu+dαv, |
for every δ≤x,y≤Δ. This last inequality and Cauchy-Schwarz inequality give
m2=(∑uv∈E(G)1)2=(∑uv∈E(G)(d2αu+d2αvdαudαv)1/2(dαudαvd2αu+d2αv)1/2)2≤∑uv∈E(G)d2αu+d2αvdαudαv∑uv∈E(G)dαudαvd2αu+d2αv≤δ−αSDDα(G)∑uv∈E(G)dαudαvdαu+dαv=δ−αSDDα(G)∑uv∈E(G)1d−αu+d−αv=δ−αSDDα(G)ISD−α(G). |
If G is a regular graph, then SDDα(G)=2m, ISD−α(G)=mδα/2 and the equality in the bound holds.
Assume now that the equality in the bound holds. Thus, by the previous argument,
1d2αu+d2αv=δ−αdαu+dαv |
for any uv∈E(G). Then Lemma 3 gives du=dv=δ for any uv∈E(G). Hence, G is a regular graph.
The modified Narumi-Katayama index is defined by
NK∗(G)=∏u∈V(G)dduu=∏uv∈E(G)dudv |
in [16], inspired in the Narumi-Katayama index [17]. Next, we present an inequality relating SDDα(G) and NK∗(G).
Theorem 5. Let G be a graph with m edges and minimum degree δ, and α>0.Then
SDDα(G)≥2δ2αmNK∗(G)−α/m, |
and the equality in the bound holds if and only if G is a regular graph.
Proof. Since the geometric mean is at most the arithmetic mean, we have
1mSDDα(G)=1m∑uv∈E(G)(dαudαv+dαvdαu)=1m∑uv∈E(G)d2αu+d2αv(dudv)α≥2δ2α1m∑uv∈E(G)1(dudv)α≥2δ2α(∏uv∈E(G)1(dudv)α)1/m=2δ2αNK∗(G)−α/m. |
If G is a regular graph, then
2δ2αmNK∗(G)−α/m=2δ2αm(δ2m)−α/m=2m=SDDα(G). |
Finally, assume that the equality in the bound holds. The previous argument gives that d2αu+d2αv=2δ2α for any uv∈E(G). Since α>0, we obtain δ2α≤d2αu,d2αv, and we have du=dv=δ for any uv∈E(G). Hence, G is regular.
Next, we obtain additional bounds of SDDα.
Theorem 6. Let G be a graph with m edges, minimum degree δ and maximum degree δ+1, α>0 and let A be the number of edges uv∈E(G) with du≠dv.Then A is an even integer and
SDDα(G)=2m+A((δ+1)αδα+δα(δ+1)α−2). |
Proof. Let F={uv∈E(G):du≠dv}, then A is the cardinality of the set F. Since the maximum degree of G is δ+1 and its minimum degree is δ, if uv∈F, then du=δ and dv=δ+1 or viceversa, and therefore
dαudαv+dαvdαu=(δ+1)αδα+δα(δ+1)α. |
If uv∈Fc=E(G)∖F, then du=dv=δ or du=dv=δ+1, and therefore
dαudαv+dαvdαu=2. |
Since there are exactly A edges in F and m−A edges in Fc, we have
SDDα(G)=∑uv∈E(G)(dαudαv+dαvdαu)=∑uv∈Fc(dαudαv+dαvdαu)+∑uv∈F(dαudαv+dαvdαu)=∑uv∈Fc2+∑uv∈F((δ+1)αδα+δα(δ+1)α)=2m−2A+A((δ+1)αδα+δα(δ+1)α). |
This gives the equality.
Seeking for a contradiction assume that A is an odd integer.
Let Γ1 be a subgraph of G obtained as follows: Γ1 is induced by the n1 vertices with degree δ in V(G); denote by m1 the number of edges of Γ1. Handshaking Lemma implies n1δ−A=2m1. Since A is an odd integer, δ is also an odd integer. Thus, δ+1 is even.
Let Γ2 be the subgraph of G induced by the n2 vertices with degree δ+1 in V(G), and denote by m2 the number of edges of Γ2. Handshaking Lemma implies n2(δ+1)−A=2m2, a contradiction, since A is odd and δ+1 is even.
Thus, we conclude that A is an even integer.
We will need the following result in the proof of Theorem 8 below.
Lemma 7. Given α>0, consider the function u:(0,∞)→(0,∞) defined as u(t)=tα+t−α.Then u strictly decreases on (0,1], u strictly increases on [1,∞) and u(t)≥u(1)=2.
Proof. We have
u′(t)=αtα−1−αt−α−1=αt−α−1(t2α−1). |
Since α>0, we have u′<0 on (0,1) and u′>0 on (1,∞). This gives the result. The following figure shows the function u(t) for some values of α.
Theorem 6 gives the precise value of SDDα when Δ=δ+1. Theorem 8 below provides a lower bound when Δ>δ+1.
Theorem 8. Let G be a graph with m edges, minimum degree δ and maximum degree Δ>δ+1. Denote by A0,A1,A2, the cardinality of the subsets of edges F0={uv∈E(G):du=δ,dv=Δ}, F1={uv∈E(G):du=δ,δ<dv<Δ}, F2={uv∈E(G):du=Δ,δ<dv<Δ}, respectively. If α>0, then
SDDα(G)≤(m−A1−A2)(Δαδα+δαΔα)+A1((Δ−1)αδα+δα(Δ−1)α)+A2(Δα(δ+1)α+(δ+1)αΔα),SDDα(G)≥2m+A0(Δαδα+δαΔα−2)+A1((δ+1)αδα+δα(δ+1)α−2)+A2(Δα(Δ−1)α+(Δ−1)αΔα−2). |
Proof. Lemma 7 gives that the function
dαvδα+δαdαv=u(dvδ) |
is increasing in dv∈[δ+1,Δ−1] and so,
(δ+1)αδα+δα(δ+1)α≤dαvδα+δαdαv≤(Δ−1)αδα+δα(Δ−1)α, |
for every uv∈F1.
In a similar way, Lemma 7 gives that the function
Δαdαv+dαvΔα=u(dvΔ) |
is decreasing in dv∈[δ+1,Δ−1] and so,
Δα(Δ−1)α+(Δ−1)αΔα≤Δαdαv+dαvΔα≤Δα(δ+1)α+(δ+1)αΔα, |
for every uv∈F2.
Also,
2≤dαudαv+dαvdαu≤Δαδα+δαΔα |
for any uv∈E(G).
We obtain
SDDα(G)=∑uv∈E(G)∖(F0∪F1∪F2)(dαudαv+dαvdαu)+∑uv∈F0(dαudαv+dαvdαu)+∑uv∈F1(dαudαv+dαvdαu)+∑uv∈F2(dαudαv+dαvdαu)≥∑uv∈E(G)∖(F0∪F1∪F2)2+∑uv∈F0(Δαδα+δαΔα)+∑uv∈F1(dαvδα+δαdαv)+∑uv∈F2(Δαdαv+dαvΔα). |
Hence,
SDDα(G)≥2m−2A0−2A1−2A2+A0(Δαδα+δαΔα)+A1((δ+1)αδα+δα(δ+1)α)+A2(Δα(Δ−1)α+(Δ−1)αΔα). |
We also have
SDDα(G)=∑uv∈E(G)∖(F1∪F2)(dαudαv+dαvdαu)+∑uv∈F1(dαudαv+dαvdαu)+∑uv∈F2(dαudαv+dαvdαu)≤(m−A1−A2)(Δαδα+δαΔα)+A1((Δ−1)αδα+δα(Δ−1)α)+A2(Δα(δ+1)α+(δ+1)αΔα). |
Here we deal with two classes of random graphs G: Erdös-Rényi (ER) graphs G(n,p) and bipartite random (BR) graphs G(n1,n2,p). ER graphs are formed by n vertices connected independently with probability p∈[0,1]. While BR graphs are composed by two disjoint sets, sets 1 and 2, with n1 and n2 vertices each such that there are no adjacent vertices within the same set, being n=n1+n2 the total number of vertices in the bipartite graph. The vertices of the two sets are connected randomly with probability p∈[0,1]. Another work in this spirit is [18].
We stress that the computational study of the variable symmetric division deg index we perform below is justified by the random nature of the graph models we want to explore. Since a given parameter set (n,p) [(n1,n2,p)] represents an infinite-size ensemble of ER graphs [BR graphs], the computation of SDDα(G) on a single graph is irrelevant. In contrast, the computation of ⟨SDDα(G)⟩ (where ⟨⋅⟩ indicates ensemble average) over a large number of random graphs, all characterized by the same parameter set (n,p) [(n1,n2,p)], may provide useful average information about the full ensemble. This computational approach, well known in random matrix theory studies, is not widespread in studies involving topological indices, mainly because topological indices are not commonly applied to random graphs; for very recent exceptions see [19,20,21,22].
From the definition of the variable symmetric division deg index, see Eq (1.1), we have that:
(i) For α=0, ⟨SDD0(G)⟩ gives twice the average number of edges of the ER graph. That is,
⟨SDD0(G)⟩=⟨∑uv∈E(G)(d0ud0v+d0vd0u)⟩=⟨∑uv∈E(G)(1+1)⟩=⟨2|E(G)|⟩=n(n−1)p. | (3.1) |
(ii) When np≫1, we can approximate du≈dv≈⟨d⟩, then
⟨SDDα(G)⟩≈⟨∑uv∈E(G)(1α+1α)⟩=⟨∑uv∈E(G)2⟩=⟨2|E(G)|⟩=n(n−1)p. | (3.2) |
(iii) By recognizing that the average degree of the ER graph model reads as
⟨d⟩=(n−1)p, | (3.3) |
we can rewrite Eq (3.2) as
⟨SDDα(G)⟩n≈⟨d⟩. | (3.4) |
We stress that Eq (3.4) is expected to be valid for np≫1.
In Figure 2(a) we plot ⟨SDDα(G)⟩ as a function of the probability p of ER graphs of size n=500. All averages in Figure 2 are computed over ensembles of 107/n random graphs. In Figure 2(a) we show curves for α∈[0,4]. The dashed-magenta curve corresponds to the case α=0, which coincides with Eq (3.1). Moreover, we observe that
⟨SDDα≤0.5(G)⟩≈⟨SDD0(G)⟩=n(n−1)p. |
However, once α>0.5, the curves ⟨SDDα(G)⟩ versus p deviate from Eq (3.1), at intermediate values of p, in the form of a bump which is enhanced the larger the value of α is. Also, in Figure 2(a) we can clearly see that Eq (3.2) is satisfied when np≫1, as expected.
Now, in Figure 2(b) we present ⟨SDDα(G)⟩ as a function of the probability p of ER graphs of three different sizes. It is clear from this figure that the blocks of curves, characterized by the different graph sizes (and shown in different colors), display similar curves but displaced on both axes. Moreover, the fact that these blocks of curves, plotted in semi-log scale, are shifted the same amount on both x− and y−axis when doubling n make us anticipate the scaling of ⟨SDDα(G)⟩. We stress that other average variable degree-based indices on ER random graphs (normalized to the graph size) have been shown to scale with the average degree [22]. Indeed, this statement is encoded in Eq (3.4), that we derived for np≫1 but should serve as the global scaling equation for ⟨SDDα(G)⟩.
Therefore, in Figure 2(c) we show ⟨SDDα(G)⟩/n as a function of the average degree ⟨d⟩ where the same curves of Figure 2(b) have been used. There we verify the global scaling of ⟨SDDα(G)⟩, as anticipated in Eq (3.4), by noticing that the blocks of curves (painted in different colors) for different graph sizes fall on top of each other.
Also, from Figure 2(a)–(c) we observe that the inequality of Theorem 1 is extended to the average variable symmetric division deg index on random graphs:
⟨SDDα(G)⟩≤⟨SDDβ(G)⟩,0<α<β; | (3.5) |
see e.g., the blue arrow in Figure 2(a) which indicates increasing α. Here, the equality is attained if and only if p=1. However, we have observed that ⟨SDDα(G)⟩≈⟨SDDβ(G)⟩ already for ⟨d⟩≥10.
In Figure 2(d), (e) we present curves of the ⟨SDDα(G)⟩ as a function of the probability p of BR graphs. For simplicity we show results for BR graphs composed by sets of equal sizes n1=n2. In Figure 2(d) we consider the case of n1=n2=500 while in (e) we report n1=n2={125,250,500}. In both figures we show curves for α∈[0,4] in steps of 0.5.
Since edges in a bipartite graph join vertices of different sets, and we are labeling here the sets as sets 1 and 2, we replace du by d1 and dv by d2 in the expression for the SDDα(G) index below. Thus,
(i) For α=0, ⟨SDD0(G)⟩ gives twice the average number of edges of the BG graph. That is,
⟨SDD0(G)⟩=⟨∑E(G)(d01d02+d02d01)⟩=⟨∑E(G)(1+1)⟩=⟨2|E(G)|⟩=2n1n2p. | (3.6) |
(ii) When both n1p≫1 and n2p≫1, we can approximate d1≈⟨d1⟩ and d2≈⟨d2⟩, then
⟨SDDα(G)⟩≈⟨∑E(G)(⟨d1⟩α⟨d2⟩α+⟨d2⟩α⟨d1⟩α)⟩=⟨|E(G)|(⟨d1⟩α⟨d2⟩α+⟨d2⟩α⟨d1⟩α)⟩. | (3.7) |
(iii) In the case we consider in Figure 2(d)–(f), where n1=n2=n/2, so that ⟨d1⟩=⟨d2⟩=⟨d⟩, Equation (3.7) reduces to
⟨SDDα(G)⟩≈⟨2|E(G)|⟩=2n1n2p=n22p. | (3.8) |
(iv) By recognizing that ⟨d⟩=np/2 we can rewrite Eq (3.8) as
⟨SDDα(G)⟩n≈⟨d⟩. | (3.9) |
We stress that Eq (3.9) is expected to be valid for np≫1. We also note that Eq (3.9) has exactly the same form as Eq (3.4).
From Figure 2(d), (e) we note that
⟨SDDα≤0.5(G)⟩≈⟨SDD0(G)⟩=2n1n2p, |
see the dashed-magenta curve in Figure 2(d). But once α>0.5, the curves ⟨SDDα(G)⟩ versus p deviate from Eq (3.6), at intermediate values of p, in the form of bumps which are enhanced the larger the value of α is. These bumps make clear the validity of inequality (3.5) on BR graphs; see e.g., the blue arrow in Figure 2(d) which indicates increasing α.
Finally, following the scaling analysis made in the previous subsection for ER graphs, in Figure 2(f) we plot the ⟨SDDα(G)⟩/n as a function of the average degree ⟨d⟩ where the same data sets of Figure 2(e) have been used. Thus we verify that ⟨SDDα(G)⟩/n scales with ⟨d⟩, as anticipated in Eq (3.9); that is, the blocks of curves (painted in different colors) for different graph sizes coincide.
In this paper we have performed analytical and computational studies of the variable symmetric division deg index SDDα(G). First, we provided a monotonicity property and obtained new inequalities connecting SDDα(G) with other well–known topological indices such as the variable inverse sum deg index, as well as the the modified Narumi-Katayama index. Then, we apply the index SDDα(G) on two ensembles of random graphs: Erd\H{o}s-Rényi graphs and bipartite random graphs. Thus, we computationally showed, for both random graph models, that the ratio ⟨SDDα(G)⟩/n is a function of the average degree ⟨d⟩ only (n being the order of the graph). We note that this last result, also observed for other variable topological indices [22], is valid for random bipartite graphs only when they are formed by sets of the same size.
Since many important topological indices can be written as
X(G)=∑uv∈E(G)F(du,dv), |
an open problem is to extend the results of this paper to other indices of this kind.
J. A. M.-B. has been supported by CONACyT (Grant No. CB-2016-286633-F). J. M. R. and J. M. S. have been supported by a grant from Agencia Estatal de Investigación (PID2019-106433GBI00/AEI/10.13039/501100011033), Spain. J. M. R. has been supported by the Madrid Government (Comunidad de Madrid-Spain) under the Multiannual Agreement with UC3M in the line of Excellence of University Professors (EPUC3M23), and in the context of the V PRICIT (Regional Programme of Research and Technological Innovation).
The authors declare there is no conflict of interest.
[1] |
Lee D, Seo J (2017) Magnetic frustration of graphite oxide. Sci Rep 7: 44690. https://doi.org/10.1038/srep44690 doi: 10.1038/srep44690
![]() |
[2] |
Novoselov KS, Geim AK, Morozov SV, et al. (2005) Two-dimensional gas of massless Dirac fermions in graphene. Nature 438: 197–200. https://doi.org/10.1038/nature04233 doi: 10.1038/nature04233
![]() |
[3] | Ohta T, Bostwick A, Seyller T, et al. (2006) Controlling the electronic structure of bilayer graphene. Science 313: 951–954. https://www.science.org/doi/10.1126/science.1130681 |
[4] |
Chen H, Müller MB, Gilmore KJ, et al. (2008) Mechanically strong, electrically conductive and biocompatible graphene paper. Adv Mater 20: 3557–3561. https://doi.org/10.1002/adma.200800757 doi: 10.1002/adma.200800757
![]() |
[5] |
Zhang Y, Tan Y-W, Stormer HL, et al. (2005) Experimental observation of the quantum hall effect and Berry's phase in graphene. Nature 438: 201–204. https://doi:10.1038/nature04235 doi: 10.1038/nature04235
![]() |
[6] |
Maher P, Dean CR, Young F, et al. (2013) Evidence for a spin phase transition at charge neutrality in bilayer graphene. Nat Phys 9: 154–158. https://doi:10.1038/nphys2528 doi: 10.1038/nphys2528
![]() |
[7] |
Huang L, Wu B, Yu G, et al. (2011) Graphene: learning from carbon nanotubes. J Mater Chem 21: 919–929. https://doi:10.1039/c0jm02225j doi: 10.1039/c0jm02225j
![]() |
[8] |
Yola ML, Eren T, Atar N (2014) A novel and sensitive electrochemical DNA biosensor based on Fe@Au nanoparticles decorated graphene oxide. Electrochim. Acta 125: 38–47. http://dx.doi.org/ 10.1016/j.electacta.2014.01.074 doi: 10.1016/j.electacta.2014.01.074
![]() |
[9] |
Gupta VK, Atar N, Yola ML (2014) A novel magnetic Fe@Au core-shell nanoparticles anchored graphene oxide recyclable nano-catalyst for the reduction of nitrophenol compounds. Water Res 48: 210–217. http://dx.doi.org/ 10.1016/j.watres.2013.09.027 doi: 10.1016/j.watres.2013.09.027
![]() |
[10] |
Yola ML, Gupta VK, Eren T, et al. (2014) A novel electro analytical nano-sensor based on graphene oxide/silver nanoparticles for simultaneous determination of quercetin and morin. Electrochim Acta 120: 204–211. http://dx.doi.org/ 10.1016/j.electacta.2013.12.086 doi: 10.1016/j.electacta.2013.12.086
![]() |
[11] |
Yola ML, Eren T, Atar N (2015) A sensitive molecular imprinted electrochemical sensor based on gold nanoparticles decorated graphene oxide: application to selective determination of tyrosine in milk. Sensor Actuat B-Chem 210: 149–157. https://doi.org/10.1016/j.snb.2014.12.098 doi: 10.1016/j.snb.2014.12.098
![]() |
[12] |
Yola ML, Atar N, Eren T, et al. (2015) Sensitive and selective determination of aqueous triclosan based on gold nanoparticles on polyoxometalate/reduced graphene oxide nanohybrid. RSC Adv 5: 65953–65962. https://doi.org/10.1039/C5RA07443F doi: 10.1039/C5RA07443F
![]() |
[13] |
Yola ML, Atar N (2017) A review: molecularly imprinted electrochemical sensors for determination of biomolecules/drug. Curr Anal. Chem 13: 13–17. https://doi.org/10.2174/1573411012666160601141018 doi: 10.2174/1573411012666160601141018
![]() |
[14] |
Yola ML, Eren T, Atar N, et al. (2016) Direct-methanol fuel cell based on functionalized graphene oxide with mono-metallic and bi-metallic nanoparticles: electrochemical performances of nanomaterials for methanol oxidation. Electroanalysis 28: 570–579. https://doi.org/10.1002/elan.201500381 doi: 10.1002/elan.201500381
![]() |
[15] |
Elçin S, Yola ML, Eren T, et al. (2016) Highly selective and sensitive voltammetric sensor based on ruthenium nanoparticle anchored calix[4]amidocrown-5 functionalized reduced graphene oxide: simultaneous determination of quercetin, morin and rutin in grape wine. Electroanalysis 28: 611–619. https://doi.org/10.1002/elan.201500495 doi: 10.1002/elan.201500495
![]() |
[16] |
Yokuş ÖA, Kardaş F, Akyıldırım O, et al. (2016) Sensitive voltammetric sensor based on polyoxometalate/reduced graphene oxide nanomaterial: application to the simultaneous determination of l-tyrosine and l-tryptophan. Sensor Actuat B-Chem 233: 47–54. http://dx.doi.org/ 10.1016/j.snb.2016.04.050 doi: 10.1016/j.snb.2016.04.050
![]() |
[17] |
Yola ML, Atar N (2016) Functionalized graphene quantum dots with bi-metallic nanoparticles composite: sensor application for simultaneous determination of ascorbic acid, dopamine, uric acid and tryptophan. J Electrochem Soc 163: B718–B725. https://dx.doi.org/10.1149/2.1191614jes doi: 10.1149/2.1191614jes
![]() |
[18] |
Yola ML, Atar N (2017) A highly efficient nanomaterial with molecular imprinting polymer: carbon nitride nanotubes decorated with graphene quantum dots for sensitive electrochemical determination of chlorpyrifos. J Electrochem Soc 164: B223–B229. https://dx.doi.org/10.1149/2.1411706jes doi: 10.1149/2.1411706jes
![]() |
[19] | Hummers WS, Offeman RE (1958) Preparation of graphitic oxide. J Am Chem Soc 80: 1339. https://pubs.acs.org/sharingguidelines |
[20] |
Alam SN, Sharma N, Kumar L (2017) Synthesis of graphene oxide by modified Hummers method and its Thermal reduction to obtain reduced graphene oxide. Graphene 6: 1–18. https://doi.org/10.4236/graphene.2017.61001 doi: 10.4236/graphene.2017.61001
![]() |
[21] |
Nyangiwe NN, Khenfouch M, Thema FT, et al. (2015) Free-green synthesis and dynamics of reduced graphene sheets via sun light irradiation. Graphene 4: 54–61. https://doi.org/10.4236/graphene.2015.43006 doi: 10.4236/graphene.2015.43006
![]() |
[22] |
Park S, An J, Potts JR. et al. (2011) Hydrazine-reduction of graphite- and graphene oxide. Carbon 49: 3019–3023. https://doi:10.1016/j.carbon.2011.02.071 doi: 10.1016/j.carbon.2011.02.071
![]() |
[23] |
Shin H-J, Kim KK, Benayad A, et al. (2009) Efficient reduction of graphite oxide by sodium borohydride and its effect on electrical conductance. Adv Funct Mater 19: 1987–1992. https://doi:10.1002/adfm.200900167 doi: 10.1002/adfm.200900167
![]() |
[24] |
Pei S, Zhao J, Du J, et al. (2010) Direct reduction of graphene oxide films into highly conductive and flexible graphene films by hydrohalic acids. Carbon 48: 4466–4474. https://doi:10.1016/j.carbon.2010.08.006 doi: 10.1016/j.carbon.2010.08.006
![]() |
[25] |
Kamat PV (1993) Photochemistry on nonreactive and reactive (semiconductor) surfaces. Chem Rev 93: 267–300. https://doi.org/10.1021/cr00017a013 doi: 10.1021/cr00017a013
![]() |
[26] |
Zhou M, Wang Y, Zhai Y, et al. (2009) Controlled synthesis of large-area and patterned electrochemically reduced graphene oxide films. Chem Eur J 15: 6116–6120. https://doi.org/10.1002/chem.200900596 doi: 10.1002/chem.200900596
![]() |
[27] |
Wang Z, Zhou X, Zhang J, et al. (2009) Direct electrochemical reduction of single-layer graphene oxide and subsequent functionalization with glucose oxidase. J Phys Chem C 113: 14071–14075. https://doi.org/10.1021/jp906348x doi: 10.1021/jp906348x
![]() |
[28] |
Wang H, Robinson JT, Li X, et al. (2009) Solvothermal reduction of chemically exfoliated graphene sheets. J Am Chem Soc 131: 9910-9911. https://doi.org/10.1021/ja904251p doi: 10.1021/ja904251p
![]() |
[29] |
Park S, Ruoff RS (2009) Chemical methods for the production of graphenes. Nat Nanotechnol 4: 217–224. https://doi.org/10.1038/nnano.2009.58 doi: 10.1038/nnano.2009.58
![]() |
[30] |
Kan E, Li Z, Yang J (2008) Magnetism in graphene system. Nano 3: 433–442. https://doi.org/10.1142/S1793292008001350 doi: 10.1142/S1793292008001350
![]() |
[31] |
Yazyev OV (2010) Emergence of magnetism in graphene materials and nanostructures. Rep Prog Phys 73: 056501. https://doi.org/10.1088/0034-4885/73/5/056501 doi: 10.1088/0034-4885/73/5/056501
![]() |
[32] |
Yazyev OV, Helm L (2007) Defect-induced magnetism in graphene. Phys Rev B 75: 125408. https://doi.org/10.1103/PhysRevB.75.125408 doi: 10.1103/PhysRevB.75.125408
![]() |
[33] | Yazyev OV, Katsnelson MI (2012) Theory of magnetism in grapheme, Advanced functional materials, Science and Technology of Atomic, Molecular, Condensed Matter & Biological Systems, Elsevier, 4: 71–103. https://dx.doi.org/10.1016/B978-0-44-453681-5.00004-2 |
[34] |
Yazyev OV, Katsnelson MI (2008) Magnetic correlations at graphene edges: Basis for novel spintronics devices. Phys Rev Lett 100: 047209. https://doi.org/10.1103/PhysRevLett.100.047209 doi: 10.1103/PhysRevLett.100.047209
![]() |
[35] |
Golor M, Wessel S, Schmidt MJ (2014) Quantum nature of edge magnetism in graphene. Phys Rev Lett 112: 46601. https://doi.org/10.1103/PhysRevLett.112.046601 doi: 10.1103/PhysRevLett.112.046601
![]() |
[36] |
Li W, Zhao M, Xia Y, et al. (2009) Covalent-adsorption induced magnetism in graphene J Mater Chem 48: 9274–9282. https://doi.org/10.1039/b908949g doi: 10.1039/b908949g
![]() |
[37] |
Santos EJG, Ayuela A, Sánchez-Portal D (2012) Universal magnetic properties of sp3-type defects in covalently functionalized graphene. New J Phys 14: 43022. https://doi.org/10.1088/1367-2630/14/4/043022 doi: 10.1088/1367-2630/14/4/043022
![]() |
[38] |
Tang T, Tang N, Zheng Y, et al. (2015) Robust magnetic moments on the basal plane of the graphene sheet effectively induced by OH groups. Sci Rep 5: 8448. https://doi.org/10.1038/srep08448 doi: 10.1038/srep08448
![]() |
[39] |
Bagani K, Bhattacharya A, Kaur J (2014) Anomalous behaviour of magnetic coercivity in graphene oxide and reduced graphene oxide. J Appl Phys 115: 023902. https://doi.org/10.1063/1.4861173 doi: 10.1063/1.4861173
![]() |
[40] |
Tang T, Liu F, Liu Y, et al. (2014) Identifying the magnetic properties of graphene oxide. Appl Phys Lett 104: 123104. https://doi.org/10.1063/1.4869827 doi: 10.1063/1.4869827
![]() |
[41] |
Lee D, Seo J, Zhu XI, et al. (2015) Magnetism in graphene oxide induced by epoxy groups. Appl Phys Lett 106: 172402. https://doi.org/10.1063/1.4919529 doi: 10.1063/1.4919529
![]() |
[42] |
Sinha A, Ali A, Thakur AD (2021) Ferromagnetism in graphene oxide. Mater Today Proceed 46: 6230–6233. https://doi.org/10.1016/j.matpr.2020.04.771 doi: 10.1016/j.matpr.2020.04.771
![]() |
[43] |
Mei X, Ouyang J (2011) Ultra sonication-assisted ultrafast reduction of graphene oxide by zinc powder at room temperature. Carbon 49: 5389–5397. https://doi.org/10.1016/j.carbon.2011.08.019 doi: 10.1016/j.carbon.2011.08.019
![]() |
[44] |
Sun P, Wang K, Wei J, et al. (2014) Magnetic transitions in graphene derivatives. Nano Res 7: 1507–1518. https://doi.org/10.1007/s12274-014-0512-1 doi: 10.1007/s12274-014-0512-1
![]() |
[45] |
Saremi S (2007) RKKY in half-filled bipartite lattices: Graphene as an example. Phys Rev B 76: 184430. https://doi.org/10.1103/PhysRevB.76.184430 doi: 10.1103/PhysRevB.76.184430
![]() |
[46] |
Wang M, Li CM (2010) Magnetism in graphene oxide. New J Phys 12: 083040. https://doi.org/10.1088/1367-2630/12/8/083040 doi: 10.1088/1367-2630/12/8/083040
![]() |
[47] |
Fujii S, Enoki T (2010) Cutting of oxidized graphene into nanosized pieces. J Am Chem Soc 132: 10034–10041. https//doi.org/10.1021/ja101265r doi: 10.1021/ja101265r
![]() |
[48] |
Boukhvalov DW (2010) Modelling of hydrogen and hydroxyl group migration on graphene. Phys Chem Chem Phys 12: 15367–15371. https://doi.org/10.1039/c0cp01009j doi: 10.1039/c0cp01009j
![]() |
[49] |
Ghaderi N, Peressi M (2010) First-principle study of hydroxyl functional groups on pristine, defected graphene, and graphene epoxide. J Phys Chem C 114: 21625–21630. https://doi.org/10.1021/jp108688m doi: 10.1021/jp108688m
![]() |
[50] |
Boukhvalov DW (2013) DFT modeling of the covalent functionalization of graphene: From ideal to realistic models. RSC Adv 3: 7150–7159. https://doi.org/10.1039/C3RA23372C doi: 10.1039/C3RA23372C
![]() |
[51] |
Santos EJG, Ayuela A, Sánchez-Portal D (2012) Universal magnetic properties of sp3-type defects in covalently functionalized graphene. New J Phys 14: 043022. https://doi.org/10.1088/1367-2630/14/4/043022 doi: 10.1088/1367-2630/14/4/043022
![]() |
[52] |
Wang M, Huang W, Chan-Park MB (2011) Magnetism in oxidized graphene with hydroxyl groups. Nanotechnology 22: 105702. https://doi.org/10.1088/0957-4484/22/10/105702 doi: 10.1088/0957-4484/22/10/105702
![]() |
[53] |
Strzelczyk R, Augustyniak-Jabtokow MA, Fedaruk R, et al. (2022) Edge ferromagnetism of graphene oxide. J Mag Mag Mater 544: 168686. https://doi.org/10.1016/j.jmmm.2021.168686 doi: 10.1016/j.jmmm.2021.168686
![]() |
[54] | Radovic LR, Bockrath B (2005) On the Chemical nature of Graphene edge: origin of stability and potential for magnetism in carbon materials. 127: 5917–5927. https://doi.org/10.1021/ja050124h |
[55] |
Ray SC, Soin N, Makgato T, et al. (2014) Graphene supported graphone/graphane bilayer nanostructure material for spintronics. Sci Rep 4: 3862. https://doi.org/10.1038/srep03862 doi: 10.1038/srep03862
![]() |
[56] |
Boukhvalov DW, Katsnelson MI (2011) sp-electron magnetic clusters with a large spin in graphene. ACS Nano 5: 2440–2446. https://doi.org/10.1021/nn103510c doi: 10.1021/nn103510c
![]() |
[57] |
Bagani K, Ray MK, Satpati B, et al. (2014) Contrasting magnetic properties of thermally and chemically reduced graphene oxide. J Phys Chem C 118: 13254–13259. https://doi.org/10.1021/jp503034d doi: 10.1021/jp503034d
![]() |
[58] |
Chuang CH, Wang Y-F, Shao Y-C, et al. (2014) The effect of thermal reduction on the photoluminescence and electronic structures of graphene oxides. Sci Rep 4: 4525. https://doi.org/10.1038/srep04525 doi: 10.1038/srep04525
![]() |
[59] |
Wang YF, Singh SB, Limaye MV, et al. (2015) Visualizing chemical states and defects induced magnetism of graphene oxide by spatially-resolved-X-ray microscopy and spectroscopy. Sci Rep 5: 15439. https://doi.org/10.1038/srep15439 doi: 10.1038/srep15439
![]() |
[60] |
Ferrari AC, Meyer JC, Scardaci V, et al. (2006) Raman spectrum of graphene and graphene layers. Phys Rev Lett 97: 187401. https://doi.org/10.1103/PhysRevLett.97.187401 doi: 10.1103/PhysRevLett.97.187401
![]() |
[61] |
Ferrari AC, Robertson J (2000) Interpretation of Raman spectra of disordered and amorphous carbon. Phys Rev B 61: 14095–14107. https://doi.org/10.1103/PhysRevB.61.14095 doi: 10.1103/PhysRevB.61.14095
![]() |
[62] |
Wang YY, Ni Zh, Yu T, et al. (2008) Raman studies of monolayer graphene: The substrate effect. J Phys Chem C 112: 10637–10640. https://doi.org/10.1021/jp8008404 doi: 10.1021/jp8008404
![]() |
[63] |
Liu Y, Tang N, Wan X, et al. (2013) Realization of ferromagnetic graphene oxide with high magnetization by doping graphene oxide with nitrogen. Sci Rep 3: 2566. https://doi.org/10.1038/srep02566 doi: 10.1038/srep02566
![]() |
[64] |
Zhou JG, Wang J, Sun CL, et al. (2011) Nano-scale chemical imaging of a single sheet of reduced graphene oxide. J Mater Chem 21: 14622–14630. https://doi.org/10.1039/c1jm11071c doi: 10.1039/c1jm11071c
![]() |
[65] |
Schniepp HC, Li JL, McAllister MJ, et al. (2006) Functionalized single graphene sheets derived from splitting graphite oxide. J Phys Chem B 110: 8535–8539. https://doi.org/10.1021/jp060936f doi: 10.1021/jp060936f
![]() |
[66] |
Shen X, Lin X, Yousefi N, et al. (2014) Wrinkling in graphene sheets and graphene oxide papers. Carbon 66: 84–92. https://doi.org/10.1016/j.carbon.2013.08.046 doi: 10.1016/j.carbon.2013.08.046
![]() |
[67] | Hua W, Gao B, Li S, et al. (2010) X-ray absorption spectra of graphene from first-principles simulations. Phys Rev B 82: 155433. https://doi.org/PhysRevB.82.155433 |
[68] |
Jeong HK, Noh H-J, Kim J-Y, et al. (2009) Comment on "Near-edge X-ray absorption fine-structure investigation of graphene". Phys Rev Lett 102: 099701. https://doi.org/http://dx.doi.org/10.1103/PhysRevLett.102.099701 doi: 10.1103/PhysRevLett.102.099701
![]() |
[69] |
Pacilé D, Papagno, Rodríguez AF, et al. (2008) Near-edge X-ray absorption fine-structure investigation of graphene. Phys Rev Lett 101: 066806. https://doi.org/10.1103/PhysRevLett.101.066806 doi: 10.1103/PhysRevLett.101.066806
![]() |
[70] |
Pacilé D, Papagno M, Rodríguez AF, et al. (2009) Comment on "Near-edge X-ray absorption fine-structure investigation of graphene". Phys Rev Lett 102: 099702. https://doi.org/10.1103/PhysRevLett.102.099702 doi: 10.1103/PhysRevLett.102.099702
![]() |
[71] |
Ganguly A, Sharma S, Papakonstantinou P, et al. (2011) Probing the thermal deoxygenation of graphene oxide using high- resolution in situ X-ray-based spectroscopies. J Phys Chem C 115: 17009–17019. https://doi.org/10.1021/jp203741y doi: 10.1021/jp203741y
![]() |
[72] |
Zhou SY, Girit ÇÖ, Scholl A, et al. (2009) Instability of two-dimensional graphene: Breaking sp2 bonds with soft X rays. Phys Rev B 80: 121409. https://doi.org/10.1103/PhysRevB.80.121409 doi: 10.1103/PhysRevB.80.121409
![]() |
[73] |
Idisi DO, Ali H, Oke JA, et al. (2019) Electronic, electrical and magnetic behaviours of reduced graphene-oxide functionalized with silica coated gold nanoparticles. Appl Surf Sci 483: 106–113. https://doi.org/10.1016/j.apsusc.2019.03.271 doi: 10.1016/j.apsusc.2019.03.271
![]() |
[74] |
Mondal A, Saha A, Sinha A, et al. (2012) Tunable catalytic performance and selectivity of a nanoparticle-graphene composite through finely controlled nanoparticle loading. Chem Asian J 7: 2931–2936. https://doi.org/10.1002/asia.201200716 doi: 10.1002/asia.201200716
![]() |
[75] |
Suda M, Kameyama N, Ikegami A, et al. (2009) Size-reduction induced ferromagnetism and photomagnetic effects in azobenzene-thiol- passivated gold nanoparticles. Polyhedron 28: 1868–1874. https://doi.org/10.1016/j.poly.2008.10.021 doi: 10.1016/j.poly.2008.10.021
![]() |
[76] |
Sarma S, Ray SC, Strydom AM (2017) Electronic and magnetic properties of nitrogen functionalized graphene-oxide. Dia Rel Mater 79: 1–6. https://doi.org/10.1016/j.diamond.2017.08.011 doi: 10.1016/j.diamond.2017.08.011
![]() |
[77] |
Ghosh B, Sarma S, Pontsho M, et al. (2018) Tuning of magnetic behaviour in nitrogenated graphene oxide functionalized with iron oxide. Dia Rel Mater 89: 35–42. https://doi.org/10.1016/j.diamond.2018.08.006 doi: 10.1016/j.diamond.2018.08.006
![]() |
[78] |
Bhattacharya G, Kandasamy G, Soin N, et al. (2017) Novel π-conjugated iron oxide/reduced graphene oxide nanocomposites for high performance electrochemical super- capacitors. RSC Adv 7: 327–335. https://doi.org/10.1039/C6RA25630A doi: 10.1039/C6RA25630A
![]() |
[79] |
Zhang X, Liu J, He B, et al. (2014) Magnetic-resonance-based electrical properties tomography: A review. IEEE Rev Biomed Eng 7: 87–96. https://doi.org/10.1109/rbme.2013.2297206 doi: 10.1109/rbme.2013.2297206
![]() |
[80] |
Carmeli I, Skakalova V, Naaman R, et al. (2002) Magnetization of chiral monolayers of polypeptide a possible source of magnetism in some biological membranes. Angew Chemie Int Ed Engl 41: 761–764. https://doi.org/10.1002/1521-3773(20020301)41:5%3C761::AID-ANIE761%3E3.0.CO;2-Z doi: 10.1002/1521-3773(20020301)41:5%3C761::AID-ANIE761%3E3.0.CO;2-Z
![]() |
[81] |
Idisi DO, Oke JA, Sarma S, et al. (2019) Tuning of electronic and magnetic properties of multifunctional r-GO-ATA-Fe2O3-composites for magnetic resonance imaging (MRI) contrast agent. J Appl Phys 126: 035301. https://doi.org/10.1063/1.5099892 doi: 10.1063/1.5099892
![]() |
[82] | Ionov AN, Volkov MP, Nikolaeva MN, et al. (2021) The magnetism of a composite based on reduced graphene oxide and polystyrene. Nanomaterials 11: 403. https://doi.org/10.3390%2Fnano11020403 |
[83] |
Cong CJ, Liao L, Liu QY, et al. (2006) Effects of temperature on the ferromagnetism of Mn-doped ZnO nanoparticles and Mn-related Raman vibration. Nanotechnology 17: 1520–1526. https://doi.org/10.1088/0957-4484/17/5/059 doi: 10.1088/0957-4484/17/5/059
![]() |
[84] | Abdelbasir S, Shalan AE (2019) Intriguing properties and applications of functional magnetic materials, In: Sahu D, Functional Materials, IntechOpen, https://doi.org/10.5772/intechopen.81386 |
[85] |
Goncalves G, Marques PAAP, Granadeiro CM, et al. (2009) Surface modification of graphene nanosheets with gold nanoparticles: The role of oxygen moieties at graphene surface on gold nucleation and growth. Chem Mater 21: 4796–4802. https://doi.org/10.1021/cm901052s doi: 10.1021/cm901052s
![]() |
[86] |
Yazyev OV, Helm L (2007) Defect-induced magnetism in graphene. Phys Rev B 75: 125408. https://doi.org/10.1103/PhysRevB.75.125408 doi: 10.1103/PhysRevB.75.125408
![]() |
[87] | Bozorth RM (1978) Ferromagnetism, Wiley-IEEE Press. |
[88] |
Sahoo PK, Panigrahy B, Li D, et al. (2013) Magnetic behaviour of reduced graphene oxide/metal nanocomposites. J Appl Phys 113: 17B525. https://doi.org/10.1063/1.4799150 doi: 10.1063/1.4799150
![]() |
[89] |
Aktürk OÜ, Tomak M (2009) AunPtn clusters adsorbed on graphene studied by first-principles calculations. Phys Rev B 80: 85417. https://doi.org/10.1103/PhysRevB.80.085417 doi: 10.1103/PhysRevB.80.085417
![]() |
[90] |
Krasheninnikov AV, Lehtinen PO, Foster AS, et al. (2009) Embedding transition-metal atoms in graphene: Structure, bonding, and magnetism. Phys Rev Lett 102: 126807. https://doi.org/10.1103/PhysRevLett.102.126807 doi: 10.1103/PhysRevLett.102.126807
![]() |
[91] | Chikazumi S, Charap SH (1964) Physics of Magnetism, New York: John Wiley & Sons. |
[92] |
Danan H, Meyer JP (1968) New determinations of the saturation magnetization of nickel and iron. J Appl Phys 39: 669. https://doi.org/10.1063/1.2163571 doi: 10.1063/1.2163571
![]() |
[93] |
Lin D, Nunes AC, Majkrzak CF, et al. (1995) Polarized neutron study of the magnetization density distribution within a CoFe2O4 colloidal particle Ⅱ. J Magn Magn Mater 145: 343. https://doi.org/10.1016/0304-8853(94)01627-5 doi: 10.1016/0304-8853(94)01627-5
![]() |
[94] |
Lu AH, Salabas EL, Schüth F, et al. (2007) Magnetic nanoparticles: Synthesis, protection, functionalization, and application. Angew Chem Int Ed 46: 1222. https://doi.org/10.1002/anie.200602866 doi: 10.1002/anie.200602866
![]() |
[95] |
Sun XC, Dong, XL (2002) Magnetic properties and microstructure of carbon encapsulated Ni nanoparticles and pure Ni nanoparticles coated with NiO layer. Mater Res Bull 37: 991. https://doi.org/10.1016/S0025-5408(02)00702-X doi: 10.1016/S0025-5408(02)00702-X
![]() |
[96] |
Matte HSSR, Subrahmanyam KS, Rao CNR (2009) Novel magnetic properties of graphene: presence of both ferromagnetic and antiferromagnetic features and other aspects. J Phys Chem C 113: 9982. https://doi.org/10.1021/jp903397u doi: 10.1021/jp903397u
![]() |
[97] |
Dutta S, Lakshmi S, Pati SK (2008) Electron-electron interactions on the edge states of graphene: A many-body configuration interaction study. Phys Rev B 77: 073412. https://doi.org/10.1103/PhysRevB.77.073412 doi: 10.1103/PhysRevB.77.073412
![]() |
[98] |
Chen Y, Peng D-L, Lin D, et al. (2007) Preparation and magnetic properties of nickel nanoparticles via the thermal decomposition of nickel organometallic precursor in alkylamines. Nanotechnology 18: 505703. https://doi.org/10.1088/0957-4484/18/50/505703 doi: 10.1088/0957-4484/18/50/505703
![]() |
[99] |
Yang X, Xia H, Qin X, et al. (2009) Correlation between the vacancy defects and ferromagnetism in graphite. Carbon 47: 1399–1406. https://doi.org/10.1016/j.carbon.2009.01.032 doi: 10.1016/j.carbon.2009.01.032
![]() |
[100] |
Novoselov KS, Geim AK, Morozov SV, et al. (2004) Electric field effect in atomically thin carbon films. Science 306: 666–669. https://doi.org/10.1126/science.1102896 doi: 10.1126/science.1102896
![]() |
[101] |
Nigar S, Zhou Z, Wang H, et al. (2017) Modulating the electronic and magnetic properties of graphene. RSC Adv 7: 51546–51580. https://doi.org/10.1039/C7RA08917A doi: 10.1039/C7RA08917A
![]() |
[102] |
Błonśki P, Tucěk J, Sofer Z, et al. (2017) Doping with graphitic nitrogen triggers ferromagnetism in graphene. J Am Chem Soc 139: 3171–3180. https://doi.org/10.1021/jacs.6b12934 doi: 10.1021/jacs.6b12934
![]() |
[103] |
Miao Q, Wang L, Liu Z, et al. (2016) Magnetic properties of N-doped graphene with high curie temperature. Sci Rep 6: 21832. https://doi.org/10.1038/srep21832 doi: 10.1038/srep21832
![]() |
[104] |
Ito Y, Christodoulou C, Nardi MV, et al. (2015) Tuning the magnetic properties of carbon by nitrogen doping of its graphene domains. J Am Chem Soc 137: 7678–7685. https://doi.org/10.1021/ja512897m doi: 10.1021/ja512897m
![]() |
[105] |
Denis PA (2022) Heteroatom co-doped graphene: The importance of nitrogen. ACS Omega 7: 45935–45961. https://doi.org/10.1021/acsomega.2c06010 doi: 10.1021/acsomega.2c06010
![]() |
[106] |
Sun P, Wang K, Wei J, et al. (2014) Magnetic transitions in graphene derivatives. Nano Res 7: 1507–1518. https://doi.org/10.1007/s12274-014-0512-1 doi: 10.1007/s12274-014-0512-1
![]() |
[107] |
Wu Y, Yu D, Feng Y, et al. (2021) Facilely synthesized N-doped graphene sheets and its ferromagnetic origin. Chinese Chem Lett 32: 3841–3846. https://doi.org/10.1016/j.cclet.2021.04.054 doi: 10.1016/j.cclet.2021.04.054
![]() |
[108] |
Talukdar N, Wang Y, Nunna BB, et al. (2021) Nitrogen-doped graphene nanomaterials for electrochemical catalysis/reactions: A review on chemical structures and stability. Carbon 185: 198–214. https://doi.org/10.1016/j.carbon.2021.09.025 doi: 10.1016/j.carbon.2021.09.025
![]() |
[109] |
Gayan ES, Soin N, Moloi SJ, et al. (2020) Polyacrylate grafted graphene oxide nanocomposites for biomedical applications. J Appl Phys 127: 054302. https://doi.org/10.1063/1.5135572 doi: 10.1063/1.5135572
![]() |
[110] |
Sarkar AK, Bediako JK, Choi J-W, et al. (2019) Functionalized magnetic biopolymeric graphene oxide with outstanding performance in water purification. NPG Asia Mater 11: 4. https://doi.org/10.1038/s41427-018-0104-8 doi: 10.1038/s41427-018-0104-8
![]() |
[111] |
Viswanathan C, Senthilkumar V, Sriranjini R, et al. (2005) Effect of substrate temperature on the properties of vacuum evaporated indium selenide thin films. Cryst Res Technol 40: 658. https://doi.org/10.1002/crat.200410404 doi: 10.1002/crat.200410404
![]() |
[112] |
Eda G, Fanchini G, Chhowalla M (2008) Large-area ultrathin films of reduced graphene oxide as a transparent and flexible electronic material. Nat Nanotechnol 3: 270. https://doi.org/10.1038/nnano.2008.83 doi: 10.1038/nnano.2008.83
![]() |
[113] |
Vozmediano MAH, López-Sancho MP, Stauber T, et al. (2005) Local defects and ferromagnetism in graphene layers. Phys Rev B 72: 155121. https://doi.org/10.1103/PhysRevB.72.155121 doi: 10.1103/PhysRevB.72.155121
![]() |
[114] |
Li G, Luican A, Lopes de Santos JMB, et al. (2010) Observation of Van Hove singularities in twisted graphene layers. Nat Phys 6: 109–113. https://doi.org/10.1038/NPHYS1463 doi: 10.1038/NPHYS1463
![]() |
[115] |
Eng AYS, Poh HL, Šaněk F, et al. (2013) Searching for magnetism in hydrogenated graphene. ACS Nano 7: 5930–5939. https://doi.org/10.1021/nn4016289 doi: 10.1021/nn4016289
![]() |
[116] |
Qin S, Guo X, Cao Y, et al. (2014) Strong ferromagnetism of reduced graphene oxide. Carbon 78: 559–565. https://doi.org/10.1016/j.carbon.2014.07.039 doi: 10.1016/j.carbon.2014.07.039
![]() |
[117] |
Taniguchi T, Yokoi H, Nagamine M, et al. (2014) Correlated optical and magnetic properties in photo-reduced graphene oxide. J Phys Chem C 118: 28258–28265. https://dx.doi.org/10.1021/jp509399x doi: 10.1021/jp509399x
![]() |
[118] |
Enayati M, Nemati A, Zarrabi A, et al. (2019) The role of oxygen defects in magnetic properties of gamma-irradiated reduced graphene oxide. J Alloys Compd 784: 134–148. https://doi.org/10.1016/j.jallcom.2018.12.363 doi: 10.1016/j.jallcom.2018.12.363
![]() |
[119] |
Enayati M, Nemati A, Zarrabi A, et al. (2018) Reduced graphene oxide: An alternative for magnetic resonance imaging contrast agent. Mater Lett 233: 363–366. https://doi.org/10.1016/j.matlet.2018.09.044 doi: 10.1016/j.matlet.2018.09.044
![]() |
[120] |
He Z, Yang X, Xia H, et al. (2011) Enhancing the ferro-magnetization of graphite by successive 12C+ ion implantation steps. Carbon 49: 1931–1938. https://doi.org/10.1016/j.carbon.2011.01.018 doi: 10.1016/j.carbon.2011.01.018
![]() |
[121] |
Soin N, Ray SC, Sarma S, et al. (2017) Tuning the electronic and magnetic properties of nitrogen functionalized few-layered graphene nanoflakes. J Phys Chem C 121: 14073. https://doi.org/10.1021/acs.jpcc.7b01645 doi: 10.1021/acs.jpcc.7b01645
![]() |
[122] |
Chuang C-H, Ray SC, Mazumder D, et al. (2017) Chemical modification of graphene oxide by nitrogenation: An x-ray absorption and emission spectroscopy study. Sci Rep 7: 42235. https://doi.org/10.1038/srep42235 doi: 10.1038/srep42235
![]() |
[123] |
Zhang K-C, Li Y-F, Liu Y, et al. (2016) Density-functional study on the structural and magnetic properties of N-doped graphene oxide. Carbon 102: 39–50. https://doi.org/10.1016/j.carbon.2016.02.030 doi: 10.1016/j.carbon.2016.02.030
![]() |
[124] |
Araki H, Yoshino K (1992) Preparation, molecular structures and novel magnetic properties of organic ferromagnetic compounds by pyrolysis of triphenoxy-triazine and benzoguanamine. J Phys Condens Matter 4: L119–L123. https://doi.org/10.1088/0953-8984/4/8/003 doi: 10.1088/0953-8984/4/8/003
![]() |
[125] |
Ganya ES, Moloi SJ, Ray SC, et al. (2020) Tuning the electronic and magnetic properties of PEDOT-PSS-coated graphene oxide nanocomposites for biomedical applications. J Mater Res 35: 2478–2490. https://doi.org/10.1557/jmr.2020.236 doi: 10.1557/jmr.2020.236
![]() |
[126] |
Ray SC, Bhunia SK, Saha A, et al. (2015) Graphene oxide (GO)/reduced-GO and their composite with conducting polymer nanostructure thin films for non-volatile memory device. Microelectron Eng 146: 48–52. https://doi.org/10.1016/j.mee.2015.04.001 doi: 10.1016/j.mee.2015.04.001
![]() |
[127] |
Eluyemi MS, Eleruja MA, Adedeji AV, et al. (2016) Synthesis and characterization of graphene oxide and reduced graphene oxide thin films deposited by spray pyrolysis method. Graphene 5: 143–154. http://dx.doi.org/ 10.4236/graphene.2016.53012 doi: 10.4236/graphene.2016.53012
![]() |
[128] |
Roy S, Soin N, Bajpai R, et al. (2011) Graphene oxide for electrochemical sensing applications. J Mater Chem 21: 14725–14731. https://doi.org/10.1039/C1JM12028J doi: 10.1039/C1JM12028J
![]() |
[129] |
Ganguly A, Sharma S, Papakonstantinou P, et al. (2011) Probing the thermal deoxygenation of graphene oxide using high-resolution in situ X-ray-based spectroscopies. J Phys Chem C 115:17009–17019. https://doi.org/10.1021/jp203741y doi: 10.1021/jp203741y
![]() |
[130] |
Roy S, Soin N, Bajpai R, et al. (2011) Graphene oxide for electrochemical sensing applications. J Mater Chem 21: 14725-14731. https://doi.org/10.1039/c1jm12028j doi: 10.1039/c1jm12028j
![]() |
[131] | Chang Y-S, Chen F-K, Tsai D-C, et al. (2021) N-doped reduced graphene oxide for room-temperature NO gas sensors. Sci Rep 11: 20719. https://doi.org/10.1038%2Fs41598-021-99883-9 |
[132] |
Singh K, Ohlan A, Saini P, et al. (2008) Poly(3, 4-ethylenedioxythiophene)γ-Fe2O3 polymer composite–super paramagnetic behavior and variable range hopping 1D conduction mechanism–synthesis and characterization. Polym Adv Technol 19: 229–236. https://doi.org/10.1002/pat.1003 doi: 10.1002/pat.1003
![]() |
[133] |
Geng D, Yang S, Zhang Y, et al. (2011) Nitrogen doping effects on the structure of graphene. Appl Surf Sci 257: 9193–9198. https://doi.org/10.1016/j.apsusc.2011.05.131 doi: 10.1016/j.apsusc.2011.05.131
![]() |
[134] |
Elk K, Richter J, Christoph V (1979) Density of states and electrical conductivity of disordered alloys with strong electron correlation. J Phys F Met Phys 9: 307–316. https://doi.org/10.1088/0305-4608/9/2/019 doi: 10.1088/0305-4608/9/2/019
![]() |
[135] |
See TP, Pandikumar A, Ngee LH, et al. (2014) Magnetically separable reduced graphene oxide/iron oxide nanocomposite materials for environmental remediation. Catal Sci Technol 4: 4396–4405. https://doi.org/10.1039/C4CY00806E doi: 10.1039/C4CY00806E
![]() |
[136] |
Ren LL, Huang S, Fan W, et al. (2011) One-step preparation of hierarchical superparamagnetic iron oxide/graphene composites via hydrothermal method. Appl Surf Sci 258: 1132–1138. https://doi.org/10.1016/j.apsusc.2011.09.049 doi: 10.1016/j.apsusc.2011.09.049
![]() |
[137] |
Tanwar S, Mathur D (2020) Magnetite-graphene oxide nanocomposites: Facile synthesis and characterization of optical and magnetic property. Mater Today Proc 30: 17–22. https://doi.org/10.1016/j.matpr.2020.03.745 doi: 10.1016/j.matpr.2020.03.745
![]() |
[138] |
Sepioni M, Nair RR, Rablen S, et al. (2010) Limits on intrinsic magnetism in graphene. Phys Rev Lett 105: 207205. https://doi.org/10.1103/PhysRevLett.105.207205 doi: 10.1103/PhysRevLett.105.207205
![]() |
[139] |
Popplewell J, Sakhnini L (1995) The dependence of the physical and magnetic properties of magnetic fluids on particle size. J Magn Mater 149: 72–78. https://doi.org/10.1016/0304-8853(95)00341-X doi: 10.1016/0304-8853(95)00341-X
![]() |
[140] |
Thapa B, Diaz-Diestra D, Badillo-Diaz D, et al. (2029) Controlling the transverse proton relaxivity of Magnetic graphene-oxide. Sci Rep 9: 5633. https://doi.org/10.1038/s41598-019-42093-1 doi: 10.1038/s41598-019-42093-1
![]() |
[141] |
Wang GS, Chen GY, Wei ZY, et al. (2013) Multifunctional Fe3O4/graphene oxide nanocomposites for magnetic resonance imaging and drug delivery. Mater Chem Phys 141: 997–1004. https://doi.org/10.1016/j.matchemphys.2013.06.054 doi: 10.1016/j.matchemphys.2013.06.054
![]() |
[142] |
Cong HP, He JJ, Lu Y, et al. (2010) Water-soluble magnetic-functionalized reduced graphene oxide sheets: situ synthesis and magnetic resonance imaging applications. Small 6: 169–171. https://doi.org/10.1002/smll.200901360 doi: 10.1002/smll.200901360
![]() |
[143] |
Zhou GM, Wang DW, Zhang LL, et al. (2010) Graphene-wrapped Fe3O4 anode material with improved reversible capacity and cyclic stability for lithium ion batteries. Chem Mater 22: 5306–5313. https://doi.org/10.1021/cm101532x doi: 10.1021/cm101532x
![]() |
[144] |
Zhang M, Lei DN, Yin XM, et al. (2010) Magnetite/graphene composites: microwave irradiation synthesis and enhanced cycling and rate performances for lithium ion batteries. Mater Chem 20: 5538–5543. https://doi.org/10.1039/C0JM00638F doi: 10.1039/C0JM00638F
![]() |
[145] |
Koo HY, Lee HJ, Go HA, et al. (2011) Graphene-based multifunctional iron oxide nanosheets with tuneable properties. Eur J 17: 1214–1219. https://doi.org/10.1002/chem.201002252 doi: 10.1002/chem.201002252
![]() |
[146] |
Ray SC, Pong WF (2022) Possible Ferro-electro-magnetic performance of "reduced graphene oxide" deposited on "ZnO-nanorod (NR) decorated with nanocrystalline (nc) Au particles". AIP Adv 12: 055008. https://doi.org/10.1063/5.0091852 doi: 10.1063/5.0091852
![]() |
[147] |
Ghosh B, Benecha EM, Ray SC, et al. (2019) ZnO nanorods decorated with nanocrystalline (nc) Au Particles: Electronic structure and magnetic behaviours. J Alloys Compd 797: 74–82. https://doi.org/10.1016/j.jallcom.2019.05.062 doi: 10.1016/j.jallcom.2019.05.062
![]() |
[148] |
Qin S, Sun P, Di Q, et al. (2015) Ferromagnetism of three-dimensional graphene framework. RSC Adv 5: 92899–92904. https://doi.org/10.1039/c5ra14377b doi: 10.1039/c5ra14377b
![]() |
[149] |
Sun Z, Yang X, Wang C, et al. (2014) Graphene activating room-temperature ferromagnetic exchange in cobalt-doped ZnO dilute magnetic semiconductor quantum dots. ACS Nano 8: 10589–10596. https://doi.org/10.1021/nn5040845 doi: 10.1021/nn5040845
![]() |
[150] |
Liu W, Speranza G (2021) Tuning the oxygen content of reduced graphene oxide and effects on its properties. ACS Omega 6: 6195–6205. https://doi.org/10.1021/acsomega.0c05578 doi: 10.1021/acsomega.0c05578
![]() |
[151] |
Chen J, Zhang W, Sun Y, et al. (2016) Creation of localized spins in graphene by ring-opening of epoxy derived hydroxyl. Sci Rep 6: 26862. https://doi.org/10.1038/srep26862 doi: 10.1038/srep26862
![]() |
[152] |
Thiyagarajan K, Muralidharan M, Sivakumar K (2018) Interfacial ferromagnetism in reduced graphene oxide-ZnO nanocomposites. J Mater Sci-Mater El 29: 7442–7452. https://doi.org/10.1007/s10854-018-8735-7 doi: 10.1007/s10854-018-8735-7
![]() |
[153] |
Ray SC, Mishra DK, Wang HT, et al. (2022) Effects of electronic structure and magnetic performance at the surface/interface of r-GO and TiO2 in r-GO/TiO2 composite thin films: X-ray absorption near-edge structure and x-ray photoelectron spectroscopy. AIP Adv 12: 075101. https://doi.org/10.1063/5.0096305 doi: 10.1063/5.0096305
![]() |
[154] |
Sarma S, Ray SC (2021) Low Temperature ferromagnetic behavior of graphene oxide (GO) and molybdenum disulphide (MoS2) hybrid nanocomposite. J Nanosci Nanotech 21: 3320–3324. https://doi.org/10.1166/jnn.2021.19286 doi: 10.1166/jnn.2021.19286
![]() |
[155] |
Ma J, Chen K (2017) Modulated self-reversed magnetic hysteresis in iron oxides. Sci Rep 7: 42312. https://doi.org/10.1038/srep42312 doi: 10.1038/srep42312
![]() |
[156] |
Yoshikazu I, Yasuhiko S (1963) Order-disorder transformation and reverse the remnant magnetism in the FeTiO3-Fe2O3 system. J Phys Chem Solids 24: 517–528. https://doi.org/10.1016/0022-3697(63)90147-1 doi: 10.1016/0022-3697(63)90147-1
![]() |
[157] |
Nord GL, Lawson CA (1992) Magnetic properties of ilmenite70–hematite30: Effect of transformation-induced twin boundaries. J Geophys Res 97: 10897–10910. https://doi.org/10.1029/91JB02259 doi: 10.1029/91JB02259
![]() |
[158] |
Hoffman KA (1992) Self-reversal of thermoremanent magnetization in the ilmenite-hematite system: Order-disorder, symmetry and spin alignment. J Geophys Res 97: 10883–10895. https://doi.org/10.1029/91JB02846 doi: 10.1029/91JB02846
![]() |
[159] | Dunin-Borkowski RE, Kasama T, Harrison RJ (2015) Electron Holography of nanostructured materials, In: Kirkland AI, Haigh SJ, Nanocharacterisation, The Royal Society of Chemistry, 2Eds., 158–210. http://dx.doi.org/10.1039/9781782621867-00158 |
[160] |
Ghosh B, Ray SC, Pattanaik S, et al. (2018) Tuning of electronic structure and magnetic properties of Xenon ion implanted zinc oxide. J Phys D-Appl Phys 51: 095304. https://doi.org/10.1088/1361-6463/aaa832 doi: 10.1088/1361-6463/aaa832
![]() |
[161] |
Matte HSSR, Maitra U, Kumar P, et al. (2012) Synthesis, characterization, and proper- ties of few-layer metal dichalcogenides and their nanocomposites with noble metal particles polyaniline, and reduced graphene oxide. ZAAC 638: 2617–2624. https://doi.org/10.1002/zaac.201200283 doi: 10.1002/zaac.201200283
![]() |
[162] |
Matte HSSR, Subrahmanyam KS, Rao CNR (2009) Novel magnetic properties of graphene: Presence of both ferromagnetic and antiferromagnetic features and other aspects. J Phys Chem C 113:9982–9985. https://doi.org/10.1021/jp903397u doi: 10.1021/jp903397u
![]() |
[163] |
Rao CNR, Matte HSSR, Subrahmanyam KS, et al. (2012) Unusual magnetic properties of graphene and related materials. Chem Sci 3: 45–52. https://doi.org/10.1039/C1SC00726B doi: 10.1039/C1SC00726B
![]() |
[164] |
Nurhafizah MD (2020) Magnetic properties of graphene oxide vis a simple mixing with waste engine oil-based carbon nanotubes. SN Appl Sci 2: 534. https://doi.org/10.1007/s42452-020-2361-8 doi: 10.1007/s42452-020-2361-8
![]() |
[165] |
El-Khawas EH, Azab AA, Mansour AM (2020) Structural, magnetic and dielectric properties of reduced graphene oxide/La0.9Bi0.1FeO3 nanocomposites. Mater Chem Phys 241: 122335. https://doi.org/10.1016/j.matchemphys.2019.122335 doi: 10.1016/j.matchemphys.2019.122335
![]() |
[166] |
Tepel M, Nesbit O, Tokmak F, et al. (1998) Sodium-dependent Cl–/HCO3– exchange in patients with chronic renal failure: correlation with renal function. Kidney Int 53: 432–438. https://doi.org/10.1046/j.1523-1755.1998.00776.x doi: 10.1046/j.1523-1755.1998.00776.x
![]() |
[167] |
Peña MA, Fierro JLG (2001) Chemical structures and performance of perovskite oxides. Chem Rev 101: 1981–2017. https://doi.org/10.1021/cr980129f doi: 10.1021/cr980129f
![]() |
[168] |
Xia Z, Poeppelmeier KR (2017) Chemistry-inspired adaptable framework structures. Acc Chem Res 50: 1222–1230. https://doi.org/10.1021/acs.accounts.7b00033 doi: 10.1021/acs.accounts.7b00033
![]() |
[169] |
Li T, Shen J, Li N, et al. (2013) Hydrothermal preparation, characterization and enhanced properties of reduced graphene-BiFeO3 nanocomposite. Mater Lett 91: 42–44. https://doi.org/10.1016/j.matlet.2012.09.045 doi: 10.1016/j.matlet.2012.09.045
![]() |
[170] |
Hu J, Wang L, Shi L, et al. (2014) Preparation of La1–xCaxMnO3 perovskite-graphene composites as oxygen reduction reaction electrocatalyst in alkaline medium. J Power Sources 269: 144–151. https://doi.org/10.1016/j.jpowsour.2014.07.004 doi: 10.1016/j.jpowsour.2014.07.004
![]() |
[171] |
Molina-García MA, Rees NV (2017) Dual-doped graphene/perovskite bifunctional catalysts and the oxygen reduction reaction. Electrochem. Commun 84: 65–70. https://doi.org/10.1016/j.elecom.2017.10.004 doi: 10.1016/j.elecom.2017.10.004
![]() |
[172] |
Dreyer DR, Park S, Bielawski CW and Ruoff RS (2010) The chemistry of graphite oxide. Chem Soc Rev 39: 228–240. https://doi.org/10.1039/B917103G doi: 10.1039/B917103G
![]() |
[173] | Nair RR, Sepioni M, Tsai IL, et al. (2012) Spin-half paramagnetism in graphene induced by point defects. Nat Phys 8: 199–202. https://www.nature.com/articles/nphys2183#Sec1 |
[174] |
Bhowmick S, Shenoy VB (2008) Edge state magnetism of single layer graphene nanostructures. J Chem Phys 128: 244717. https://doi.org/10.1063/1.2943678 doi: 10.1063/1.2943678
![]() |
[175] |
Chen L, Guo L, Li Z, et al. (2013) Towards intrinsic magnetism of graphene sheets with irregular zigzag edges. Sci Rep 3: 2599. https://doi.org/10.1038/srep02599 doi: 10.1038/srep02599
![]() |
[176] |
López-Sancho MP, De Juan F, Vozmediano MAH (2009) Magnetic moments in the presence of topological defects in graphene. Phys Rev B-Condens Matter Mater Phys 79: 075413. https://doi.org/10.1103/PhysRevB.79.075413 doi: 10.1103/PhysRevB.79.075413
![]() |
[177] |
Krishnamoorthy K, Veerapandian M, Yun K, et al. (2013) The chemical and structural analysis of graphene oxide with different degrees of oxidation. Carbon 53: 38–49. https://doi.org/10.1016/j.carbon.2012.10.013 doi: 10.1016/j.carbon.2012.10.013
![]() |
[178] |
Thompson-Flagg RC, Moura MJB, Marder M (2009) Rippling of graphene. Epl 85: 46002. https://doi.org/10.1209/0295-5075/85/46002 doi: 10.1209/0295-5075/85/46002
![]() |
[179] |
Bagani K, Bhattacharya A, Kaur J, et al. (2014) Anomalous behaviour of magnetic coercivity in graphene oxide and reduced graphene oxide. J Appl Phys 115: 023902. https://doi.org/10.1063/1.4861173 doi: 10.1063/1.4861173
![]() |
[180] |
Kumazaki H, Hirashima D (2008) Nonmagnetic-defect-induced magnetism in graphene. Physica E 40: 1703–1705. https://doi.org/10.1016/j.physe.2007.10.112 doi: 10.1016/j.physe.2007.10.112
![]() |
[181] |
Li W, Zhao M, Xia Y, et al. (2009) Covalent adsorption induced magnetism in graphene. J Mater Chem 19: 9274–9282. https://doi.org/10.1039/B908949G doi: 10.1039/B908949G
![]() |
[182] |
Tang T, Liu F, Liu Y, et al. (2014) Identifying the magnetic properties of graphene oxide. Appl Phys Lett 104/12: 123104. https://doi.org/10.1063/1.4869827 doi: 10.1063/1.4869827
![]() |
[183] |
Bedanta S, Kleemann W (2009) Topical review supermagnetism. J Phys D-Appl Phys 42: 013001. https://doi.org/10.1088/0022-3727/42/1/013001 doi: 10.1088/0022-3727/42/1/013001
![]() |
[184] |
Fujii S, Enoki T (2010) Cutting of oxidized graphene into nanosized pieces. J Am Chem Soc 132: 10034–10041. https://doi.org/10.1021/ja101265r doi: 10.1021/ja101265r
![]() |
[185] |
Hernández Rosas JJ, Ramírez Gutiérrez RE, Escobedo-Morales A, et al. (2011) First principles calculations of the electronic and chemical properties of graphene, graphane, and graphene oxide. J Mol Model 17: 1133–1139. https://doi.org/10.1007/s00894-010-0818-1 doi: 10.1007/s00894-010-0818-1
![]() |
[186] |
Li W, Zhao M, Xia Y, et al. (2009) Covalent-adsorption induced magnetism in graphene. J Mater Chem 19: 9274–9282. https://doi.org/10.1039/b908949g doi: 10.1039/b908949g
![]() |
1. | Bahati Erick, Maranya Mayengo, Modelling the dynamics of Cassava Mosaic Disease with non-cassava host plants, 2022, 33, 23529148, 101086, 10.1016/j.imu.2022.101086 | |
2. | Caihong Song, Ning Li, Dynamic analysis and bifurcation control of a fractional-order cassava mosaic disease model, 2022, 1598-5865, 10.1007/s12190-022-01809-9 | |
3. | Nabeela Anwar, Shafaq Naz, Muhammad Shoaib, Reliable numerical treatment with Adams and BDF methods for plant virus propagation model by vector with impact of time lag and density, 2022, 8, 2297-4687, 10.3389/fams.2022.1001392 | |
4. | Geofrey Sikazwe, Rosita E.E. Yocgo, Pietro Landi, David M. Richardson, Cang Hui, Managing whitefly development to control cassava brown streak virus coinfections, 2024, 493, 03043800, 110753, 10.1016/j.ecolmodel.2024.110753 | |
5. | Tariq Q. S. Abdullah, Gang Huang, Wadhah Al-Sadi, Yasser Aboelmagd, Wael Mobarak, Fractional Dynamics of Cassava Mosaic Disease Model with Recovery Rate Using New Proposed Numerical Scheme, 2024, 12, 2227-7390, 2386, 10.3390/math12152386 | |
6. | Sireepatch Sangsawang, Usa Humphries, Amir Khan, Puntani Pongsumpun, Sensitivity analysis and optimal control for the dynamic mathematical model of cassava mosaic disease, 2024, 14, 2158-3226, 10.1063/5.0207497 | |
7. | E. Azroul, N. Kamali, M. Shimi, Novel insights into Cassava mosaic disease using Caputo fractional derivative: modeling and analysis, 2025, 0003-6811, 1, 10.1080/00036811.2025.2509314 |