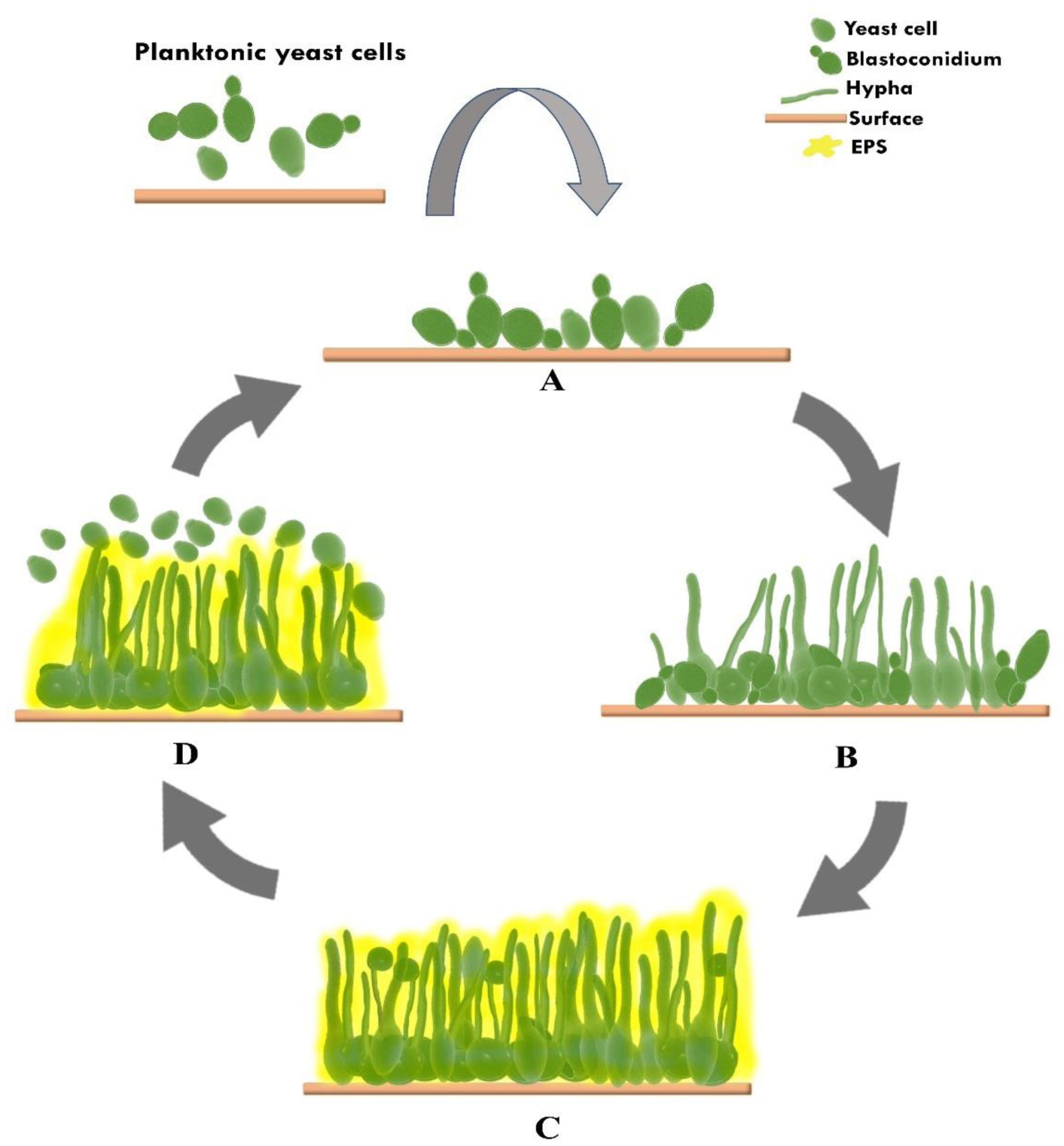
This study investigated the risk-return relationship using the Capital Asset Pricing Model (CAPM), Carhart four-factor Model (C4FM) and Fama and French Multifactor Models (FFMMs): F3FM and F5FM. This study analyzed the JSE ALSI returns of the South African market, in relation to the risk factors constructed by the data of twenty-six emerging markets, over the sample period from October 2000 to October 2021. The methodology employed was a comparison of the conventional regression analysis and novel Bayesian approach. Regression analysis estimated by Ordinary Least Squares (OLS) is one of the foremost methods used in South Africa. However, such an approach does not take into account the properties of the price data, namely, the asymmetric, volatile and random nature. While the Newey-West adjustment is one way to assist in capturing autocorrelation and volatility, it fails to consider asymmetry. The Bayesian approach accounts for all the aforementioned properties, overcoming the fundamental flaws of regression analysis. The additional model diagnostics highlighted in this study improved the Bayesian approach' robustness. The risk factors of the FFMMs estimated by regression analysis, with and without the Newey-West adjustment, were insignificant, whereas the Bayesian test results were significant. This finding clearly highlighted that model choice impacts the significance of parameter estimation and the financial decisions of investors, firms and policymakers. Jensen's alpha revealed CAPM to be optimal but none of the asset pricing models fully captured the risk premia. Thus, returns should be investigated with different risk measures for future research purposes.
Citation: Nitesha Dwarika. Asset pricing models in South Africa: A comparative of regression analysis and the Bayesian approach[J]. Data Science in Finance and Economics, 2023, 3(1): 55-75. doi: 10.3934/DSFE.2023004
[1] | Patrick Di Martino . Extracellular polymeric substances, a key element in understanding biofilm phenotype. AIMS Microbiology, 2018, 4(2): 274-288. doi: 10.3934/microbiol.2018.2.274 |
[2] | McKenna J. Cruikshank, Justine M. Pitzer, Kimia Ameri, Caleb V. Rother, Kathryn Cooper, Austin S. Nuxoll . Characterization of Staphylococcus lugdunensis biofilms through ethyl methanesulfonate mutagenesis. AIMS Microbiology, 2024, 10(4): 880-893. doi: 10.3934/microbiol.2024038 |
[3] | Davide Gerardi, Sara Bernardi, Angelo Bruni, Giovanni Falisi, Gianluca Botticelli . Characterization and morphological methods for oral biofilm visualization: where are we nowadays?. AIMS Microbiology, 2024, 10(2): 391-414. doi: 10.3934/microbiol.2024020 |
[4] | Luciana C. Gomes, Filipe J. Mergulhão . Effect of heterologous protein expression on Escherichia coli biofilm formation and biocide susceptibility. AIMS Microbiology, 2016, 2(4): 434-446. doi: 10.3934/microbiol.2016.4.434 |
[5] | Joseph O. Falkinham . Mycobacterium avium complex: Adherence as a way of life. AIMS Microbiology, 2018, 4(3): 428-438. doi: 10.3934/microbiol.2018.3.428 |
[6] | Zoya Samoilova, Alexey Tyulenev, Nadezhda Muzyka, Galina Smirnova, Oleg Oktyabrsky . Tannic and gallic acids alter redox-parameters of the medium and modulate biofilm formation. AIMS Microbiology, 2019, 5(4): 379-392. doi: 10.3934/microbiol.2019.4.379 |
[7] | Ruba Mirghani, Tania Saba, Hebba Khaliq, Jennifer Mitchell, Lan Do, Liz Chambi, Kelly Diaz, Taylor Kennedy, Katia Alkassab, Thuhue Huynh, Mohamed Elmi, Jennifer Martinez, Suad Sawan, Girdhari Rijal . Biofilms: Formation, drug resistance and alternatives to conventional approaches. AIMS Microbiology, 2022, 8(3): 239-277. doi: 10.3934/microbiol.2022019 |
[8] | Phu-Tho Nguyen, Tho-Thi Nguyen, Duc-Cuong Bui, Phuoc-Toan Hong, Quoc-Khanh Hoang, Huu-Thanh Nguyen . Exopolysaccharide production by lactic acid bacteria: the manipulation of environmental stresses for industrial applications. AIMS Microbiology, 2020, 6(4): 451-469. doi: 10.3934/microbiol.2020027 |
[9] | Manuela Oliveira, Eva Cunha, Luís Tavares, Isa Serrano . P. aeruginosa interactions with other microbes in biofilms during co-infection. AIMS Microbiology, 2023, 9(4): 612-646. doi: 10.3934/microbiol.2023032 |
[10] | Stephen T. Abedon . Phage “delay” towards enhancing bacterial escape from biofilms: a more comprehensive way of viewing resistance to bacteriophages. AIMS Microbiology, 2017, 3(2): 186-226. doi: 10.3934/microbiol.2017.2.186 |
This study investigated the risk-return relationship using the Capital Asset Pricing Model (CAPM), Carhart four-factor Model (C4FM) and Fama and French Multifactor Models (FFMMs): F3FM and F5FM. This study analyzed the JSE ALSI returns of the South African market, in relation to the risk factors constructed by the data of twenty-six emerging markets, over the sample period from October 2000 to October 2021. The methodology employed was a comparison of the conventional regression analysis and novel Bayesian approach. Regression analysis estimated by Ordinary Least Squares (OLS) is one of the foremost methods used in South Africa. However, such an approach does not take into account the properties of the price data, namely, the asymmetric, volatile and random nature. While the Newey-West adjustment is one way to assist in capturing autocorrelation and volatility, it fails to consider asymmetry. The Bayesian approach accounts for all the aforementioned properties, overcoming the fundamental flaws of regression analysis. The additional model diagnostics highlighted in this study improved the Bayesian approach' robustness. The risk factors of the FFMMs estimated by regression analysis, with and without the Newey-West adjustment, were insignificant, whereas the Bayesian test results were significant. This finding clearly highlighted that model choice impacts the significance of parameter estimation and the financial decisions of investors, firms and policymakers. Jensen's alpha revealed CAPM to be optimal but none of the asset pricing models fully captured the risk premia. Thus, returns should be investigated with different risk measures for future research purposes.
Biofilm is an attachment complex consisting of an assemblage of microorganism cells with a three-dimensional structure on a particular surface, and it occurs irreversibly. This attachment can occur on biotic (the host tissue) or abiotic surfaces (medical devices). The structural matrix can adhere to and be embedded in the surface due to microorganisms' extracellular polymeric substances (EPSs). One of the microorganisms that can produce biofilms to protect against the environment is Candida yeast, especially Candida albicans (C. albicans) [1],[2]. The formation of Candida biofilms is often a result of a source of nosocomial infections related to candidemia in intensively hospitalized patients because of its ability to grow to become attached to medical devices or host tissues [3],[4].
Candida, the most common cause of invasive fungal infection in hospitalized patients, has mortality rates that vary depending on the study population but are consistently high worldwide. In the case of neonates, the mortality rate reaches 35–60% [5], while, in pediatrics, it ranges between 10–35% [6], and 19.6–67% in adults [7],[8]. Candida is the primary agent of fungal sepsis, with a prevalence of 10–15%, and with 5% having severe sepsis or septic shock [9]. Among patients with septic shock, candidemia is the clinical condition with the highest mortality, ranging from 54 to 66% [10],[11]. The survival of patients with sepsis due to candidemia is strongly related to the early source control of medical devices used and appropriate antifungal treatment.
Candida bloodstream infection (CBSI) associated with catheter use has a high mortality rate, particularly in prolonged hospital stays in intensive care unit (ICU) patients [3],[12],[13]. The main culprit of CBSI related to catheter use is microbial biofilm formation [1],[14],[15]. Therefore, this review will focus on the discussion of the Candida biofilm formation mechanisms, especially in C. albicans, which is the primary source of Candida catheter-related bloodstream infection (CRCBSI), as well as the characteristics of biofilms by various non-albicans Candida (NAC) species, as well as mixed biofilms, and the principles of appropriate management and prevention.
CBSI, also called candidemia, is the most common form of invasive fungal disease with Candida present in the blood [10],[12]. Several factors have been associated with the risk of candidemia in prolonged hospital stays in ICU patients, including using a catheter, total parenteral nutrition, systemic broad-spectrum antibiotic usage, Candida colonization history, history of surgery and being in immunosuppressive therapy. Moreover, underlying factors include immunocompromised persons with co-morbidities such as malignancy and hematological disorders, premature infants and low-birth-weight neonates; solid organ transplantation, neutropenia, extensive burns and renal dysfunction have also been reported as predispositions to CBSI [3],[12],[13],[16].
The epidemiology of candidemia varies according to geography, although it is dominated mainly by C. albicans, with the number of NAC infections increasing yearly [17]. Most invasive infections caused by NAC comprise C. tropicalis and C. glabrata, followed by C. parapsilosis and C. krusei [18]. Candida albicans and C. parapsilosis are the most frequent biofilm producers because of their ability of morphogenesis, facilitating the persistent infection associated with intravascular catheter use [19].
The CBSI related to peripheral intravascular and central venous catheters (CVC) is referred to as CRCBSI, with more than 90% being due to a CVC. The most frequent causative agents of CRCBSI are gram-positive bacteria, gram-negative bacteria and Candida yeasts [19],[20]. Catheters commonly used in ICU patients increase the risk of biofilm formation, which can lead to candidemia [15],[17],[19],[21]. Candidemia catheter-related infection has a high morbidity and mortality rate because it is associated with nosocomial infection, with higher mortality than bacterial biofilms, although it only accounts for about 5–10% of all sepsis cases and the incidence is rarely reported. In most cases, these lower prevalences caused by the classic diagnostic criteria used for sepsis and septic shock do not meet candidemia criteria [9],[11],[22]. Interestingly, Bassetti et al. [10] demonstrated that the use of a CVC is less commonly associated with intra-abdominal infection. These results support the observation of a recent murine model experiment that showed an increase in the number of Candida in the gut is associated with increased severity of bacterial sepsis due to the possibility of induction of a cytokine storm and/or decreased macrophage-killing activity [23].
Candida albicans is the most common commensal species isolated for candidemia, with high mortality rates being observed despite appropriate antifungal drug treatment [1],[3],[15],[24],[25]. The human fungal opportunistic pathogen C. albicans is a dimorphic fungus that can change morphologically from budding yeast to filamentous pseudohyphae and hyphae. This ability to undergo morphogenesis in the host helps it to adhere to or attack and form biofilms that contribute as virulence factors [26]–[28]. It also has a remarkable ability to form chlamydospores, which are thick-walled cells at the end of hyphae [29]. The formation of the C. albicans biofilm includes four main stages (Figure 1).
The first is the attachment process of C. albicans planktonic yeast cells to surfaces such as the epithelium, biomaterials or cellular aggregates [1],[25]. Furthermore, yeast cells will proliferate to form microcolonies and a basal layer of biofilm [25],[30]. The first phase in vitro takes approximately 11 hours. In comparison, in vivo experiments in a rat CVC have a shorter duration of 8 hours [1],[31]. The attachment process (Figure 1A) is mediated by fungal cell wall proteins called adhesins from three families, i.e., the agglutinin-like sequence (Als) family, the hyphal wall protein (Hwp) family and the individual protein file family F/hyphally regulated (Iff/Hyr) family [1],[4],[24],[32],[33]. Adhesins promote attachment to other cells, e.g., epithelial or microbial cells, or abiotic surfaces, by binding to specific amino acids or carbohydrate residues [1],[25]. The Als adhesin family of C. albicans consists of eight members, namely, Als1–7 and 9, whose proteins have a similar structure and contain N-terminal secretory signaling sequences. In C. albicans, Als1, Als3 and Als5 were most involved in the attachment process to surface and host cells [25],[32]–[34]. The Hwp adhesin families are required for biofilm development, including Hwp1 and Hwp2, as well as for enhanced adherence to polystyrene 1 (Eap1), which is repressed by Tup1 (Rbt1), and yeast wall protein (Ywp1) (Table 1). Hwp 1 is a major hyphal cell wall protein and the most studied cell wall mannoprotein in C. albicans germ tube form and hyphae, which promote attachment to the host surface [25],[32],[33]. In addition, once attachment occurs, persister cells that are phenotypic variants of wild-type cells and constitute only a tiny proportion of the biofilm population will also form, although their proportion will remain constant during biofilm development. These cells are highly associated with cases of remarkable tolerance because they do not grow but can survive high doses of antifungal treatment [35].
The second stage is the increase in the number of yeast cells (proliferation), and then the transitional stage or morphological modification, where yeast cells change into hyphae (Figure 1B) [1],[25]. Biofilm development in this stage involves the transcriptional regulators: Bcr1, Brg1, Efg1, Tec1, Ndt80 and Rob1 (Table 1) [24],[32],[33]. The attachment of hyphae is specifically mediated by Hwp1, a gene that is required for hyphal development, mating and the maintenance of biofilm integrity [36]. The hypha form has an essential contribution to the overall architectural stability of the biofilm and acts as a supporting scaffold for fellow yeast cells, pseudohyphae, hyphae and other microbial cells in the context of polymicrobial biofilms. Thus, this morphogenesis in C. albicans and the ability of these hyphae to adhere to one another is very important for developing and maintaining biofilms [28],[33],[36].
The third stage is the maturation process, in which fungal cells called sessile cells become organized and trapped in the EPS, where they produce to form the three-dimensional architectural matrix of the mature biofilm structure (Figure 1C) [37]. The EPS has a hydrated, gel-like three-dimensional structure, immobilizing some biofilm cells to support structural integrity [1],[37]. The extracellular matrix (ECM) formed is an organic polymer consisting of proteins (55%), carbohydrates (25%), lipids (15%) and extracellular deoxyribonucleic acid (eDNA) (5%) [15],[24],[25],[37]. There are more than 500 proteins identified in the biofilm matrix, with the majority of glycolysis enzymes that may degrade extracellular biopolymers acting as a source of energy and heat shock protein (Hsp) [24],[37]. The dominant polysaccharide in ECM biofilms of C. albicans is the mannans glucan complex, namely, ∝-1,2 branched ∝-1,6 mannan, which is associated with linear β-1,6 glucan and a small amount of β-1,3 glucan. Although β-1,3 glucan is only a small component, it plays a significant role in biofilm resistance to antifungals, especially azole groups, by inhibiting drug diffusion. The profile lipid of the ECM is composed of glycerolipids (99.5%), for much fewer sphingolipids (0.5%). In addition, the only sterol detected was ergosterol, though at low concentrations [37]. Another major contributor to the stability and growth of C. albicans biofilms in the ECM is eDNA with predominantly random non-coding sequences. The proposed mechanisms for releasing eDNA into the matrix include cell lysis, quorum sensing (QS) and excretion from DNA-containing vesicles [32],[37]. The QS also mediates communication between sessile cells in the morphogenesis and biofilm formation of C. albicans. The molecule of QS that plays a role is tyrosol, which induces filamentation and biofilm formation, while farnesol plays the opposing role [38].
The ECM is partially self-produced and secreted by C. albicans cells within the biofilm but may also contain aggregates from the surrounding environment. These aggregates include C. albicans cells and lysed host cells, as well as host-specific cells recruited to the region and fused into biofilms, such as epithelial cells and neutrophils. Therefore, the composition of the ECM may vary depending on the location of the biofilm in the host [2]. The ECM's unique structure can protect innate host immune defenses, especially against neutrophils and monocytes [25],[37]. Monocytes can phagocytize C. albicans planktonic cells, but not sessile cells in biofilms. Furthermore, neutrophils cannot eliminate mature biofilms due to a failure to release extracellular traps, as well as failed activation due to the inhibition of matrix glucans, which act as decoy mechanisms and cannot activate reactive oxygen species [2],[4],[37]. Two transcription factors involved in regulating ECM production for C. albicans biofilms is Zap1 and Rlm1 (Table 1) [32],[33].
The ECM in biofilms also plays an essential role in antifungal resistance, although it is multifactorial and based on different mechanisms. One of the mechanism is demonstrated by the dominant role of component β-1,3 glucan in biofilm resistance to fluconazole and amphotericin B due to sequestration of antifungal matrix components that prevent the drug from reaching cellular targets [37],[39]–[41]. Another matrix component that plays a role is eDNA; Martins et al. [42] reported that the addition of DNase increased the susceptibility of some antifungals, such as echinocandins and polyenes, but not fluconazole.
The fourth stage is the dispersal of mature biofilms (Figure 1D) by releasing budding daughter yeast cells as the elongated cells that are not attached to colonize other parts to form a secondary infection site [1],[15],[30],[38]. Detachment of yeast-like cell buds from the hyphae's upper biofilm layer allows C. albicans to disseminate to the bloodstream and cause invasive disease. Although the released cells resemble yeast, they have a unique transcriptional profile and differ from planktonic cells or biofilms by showing increased virulence and causing more damage to endothelial cells [15],[30]. These cells are believed to play a significant role in the pathogenesis of vascular catheters and other infections that cause disseminated disease [15]. The genes that regulate the process of dispersal of yeast cells are Ume6, Pes1 and Nrg1 (Table 1) [32],[33].
Gene name | Description | Stage |
Als | Consists of eight large cell surface glycoproteins: Als1–7,9; with Als 1, Als3 and Als5 as the most involved in the attachment process | Attachment |
Hwp | Hwp1, Hwp2 and Rbt1 (repressed by Tup1) belong to this gene family, which is required for adhesion to host cell surface proteins and cell aggregationHwp1 is also required for hyphal formation | Attachment and transitional |
Bcr1 | Important in the adhesion process of yeast cells to the surface, and also acts as a trancription factor for upregulation in Hwp1 expression | Attachment and transitional |
Efg1 | ||
Brg1 | Transcription factor to promote hyphal formation | Transitional |
Rob1 | ||
Tec1 | ||
Ndt80 | ||
Zap1 | Negative regulator of ECM production | Maturation |
Rlm1 | Positive regulator of ECM production | |
Nrg1 | Overexpression leads to increased dispersion cells | Dispersion |
Pse1 | ||
Ume6 | Overexpression leads to reduced dispersion cells | Dispersion |
The formation of biofilms in Candida spp. is different in the morphology, characteristics of the ECM and their ability of antifungal resistance [29],[43]. The most pathogenic species often found to form biofilms is C. albicans, which will be discussed in the formation mechanism. Furthermore, NAC and some species formerly known as genus Candida, are important in cases of candidemia and have the ability to form biofilms, including C. tropicalis, C. parapsilosis, C. dubliniensis, C. auris, Nakaseomyces glabrata (also called C. glabrata), Pichia kudriavzevii (also called C. krusei) and Meyerozyma guilliermondii (also called C. guilliermondii) [29],[43]–[45]. Nonetheless, the exact mechanism of these NACs and their morphogenesis on pathogenicity in biofilm formation has not been fully characterized. The formation of biofilms in Candida spp. is different in terms of morphology, characteristics of the ECM and their ability of antifungal resistance [29],[43]. The differences in these biofilms' structures and the ECMs' composition are affected by the environmental conditions experienced by Candida spp. One crucial factor is the substrate of the abiotic surface to which the biofilm is attached. Several substrates are used to study Candida biofilms in vivo and in vitro, such as polystyrene, polyurethane, polymethylmethacrylate, polyvinyl chloride (PVC), cellulose, silicone and latex elastomer [1].
Candida tropicalis has emerged as the second or third most common and virulent agent of candidemia, and it is particularly relevant in catheter-associated urinary tract infections with high biofilm-forming capacity [1],[3],[44]. The biofilm structure is composed of a dense network of yeast cells, pseudohyphae and true hyphae enclosed in an ECM [33],[43]. Candida tropicalis is also a robust and efficient biofilm producer of the EPS's high thickness [44]. The ECM composition consists of low carbohydrate and protein levels, hexosamine, phosphorus and uronic acid [1],[33],[44]. The gene that plays a role in the adhesion process is ALS1–16, while the maturation process is carried out with Efg1 [33]. Candida tropicalis can produce biofilms significantly on silicon, PVC and polyurethane catheters, and it is reported to be resistant to azoles, especially to fluconazole [44],[46],[47].
The increasing use of medical devices and parenteral nutrition has made C. parapsilosis the most frequently isolated NAC fungal organism associated with catheter use, especially in critically ill neonates and transplant recipients [48]–[51]. The hypothesis of a high rate of C. parapsilosis infection in neonates is thought to be associated with its ability to utilize fat and fatty acids as the primary energy source in critically ill patients; it is believed to be capable of growing in a solution with high glucose concentration, especially in neonates who often receive parenteral nutrition [49],[50],[52]. This fungus is also the causative agent of several catheter-related infections due to being frequently found on the skin of healthy humans [48],[53]. Their characteristics and ability to switch into pseudohyphae and form biofilms on prosthetic materials, such as CVCs, could create nosocomial outbreaks with high mortality. Candida parapsilosis biofilm consists of clumping blastoconidia, yeast cells and pseudohyphae, with a thin ECM consisting of high carbohydrate levels with low protein. The formation of a C. parapsilosis biofilm is best demonstrated on a PVC catheter. Several genes involved in forming the C. parapsilosis biofilm were also found in the C. albicans, such as Bcr1, Efg1 and Hwp1 [1],[33]. Moreover, C. parapsilosis isolates have been found to increase resistance to azoles, especially fluconazole, at a rate five times higher than in C. albicans; they also have intrinsic resistance to echinocandins [54].
Candida dubliniensis is a species with many similar phenotypic characteristics to C. albicans, and it was often misidentified due to its ability to form germ tubes, chlamydospores and hyphae, but with fewer virulence genes than C. albicans [55],[56]. These two types of Candida are also known to secrete farnesol, a QS molecule that inhibits Candida biofilm development through the inhibition of filamentation, which in turn may decrease its potential to invade deeper tissues [1],[57]. Nonetheless, genes involved in biofilm formation have not yet been explored. In addition, C. dubliniensis is much less common in the microbiome, which correlates with the low prevalence of C. dubliniensis as invasive candidiasis [55],[58]. The differences between the two species include the following: C. dubliniensis can grow well at 30 °C and 37 °C, like C. albicans in Sabouraud dextrose agar culture media; however, it does not grow at all at 42 °C [55]. The prevalence of isolates of this species is very high in the oral cavity of patients infected with human immunodeficiency virus (HIV) or acquired immune deficiency syndrome (AIDS), probably due to its increased ability to adhere to buccal epithelial cells. However, the underlying reason is unclear [55],[56],[58]–[60]. Candida dubliniensis has also shown resistance to fluconazole, due to its increased use as prophylaxis in HIV/AIDS patients [54],[55],[60].
Candida auris survives well on fomites, medical equipment and surfaces in the hospital environment with chlorhexidine clearance [61]–[63]. A characteristic of C. auris in biofilms is that it is also less sensitive to several disinfectants, such as hydrogen peroxide, povidone-iodine and sodium hypochlorite [45]. This fungus can also spread between patients, mainly in hospitalized patients with severe illness, or with the prolonged use of indwelling devices, thus later causing candidemia with high mortality. The C. auris biofilms consist primarily of budding yeast cells, which are round/oval with a small ECM; and, pseudohyphae are rarely found [64]. The ECM component of C. auris contains many mannan-glucan polysaccharides [45],[62]. Along with its discovery and characteristics, it has become an epidemic in several healthcare centers globally, which is very important because it has multidrug-resistant properties to antifungals [61],[65]. Resistance has been demonstrated, primarily to fluconazole, with variable susceptibility to other azoles, amphotericin B and echinocandins [45],[54],[63].
Nakaseomyces glabrata (C. glabrata) is some regions' second most common cause of invasive disseminated candidiasis; it is a poor biofilm producer in a catheter, but is strong in oral isolates [18],[66],[67]. It also showed to form biofilms on different medical devices [68]–[70]. These fungi are not dimorphic due to the inability of this species to form filaments of either true hyphae or pseudohyphae as commensals or pathogens; thus, the formed biofilm consists of only monolayer or multilayer blastoconidia that are tightly packed and wrapped in a thin ECM [1],[29],[33]. Despite that, C. glabrata has an ECM matrix component with high carbohydrate and protein content. The adherence mechanism is mediated by epithelial adhesins that have a similar structure to the Als proteins [70]. Estivill et al. [47] showed that C. glabrata biofilm formation was best on PVC. Furthermore, many C. glabrata strains that cause septicemia are resistant to antifungal azoles, particularly fluconazole, and strains with mutations in the FKS gene show resistance to echinocandins [1],[54].
Pichia kudriavzevii (C. krusei) is often found in patients with underlying hematological malignancy [18]. This fungus can produce intermediate biofilms on PVC and polyethylene surfaces [29],[71]. Unfortunately, the significance of C. krusei biofilms and their morphogenesis for pathogenicity has not been fully characterized. Like C. albicans, C. krusei has the property of thermomorphism, that is, it can change the shapes of blastoconidia and pseudohyphae into hyphae at 37 °C so that the primary regulatory network that regulates dimorphism related to biofilm formation is also similar [71]. Candida krusei is intrinsically resistant to fluconazole, and infections due to this species commonly occur in patients receiving fluconazole prophylaxis and who have a prior history of neutropenia [18],[71].
Biofilm infection can be caused by a single microbial species or a mixture of different species, but more studies have focused on a single species in various host niches [25],[72],[73]. Polymicrobial interactions can alter the expression of virulence factors; thus, the biofilms that form are often more resistant to antimicrobial drugs [72]. In addition, polymicrobial interactions can also affect the host's immune response and the outcome of infection because the immune response to one species can affect immunity to other organisms [72],[73]. The interaction between Candida spp., especially C. albicans with microbes found on biotic and abiotic surfaces, which are very varied and complex, consists of synergistic or antagonistic relationships [25],[72]–[74]. The interaction of C. albicans with other microorganisms can occur through coaggregation and coadhesion processes [74].
Candida polymicrobial biofilms can associate synergistically or antagonistically with gram-positive and gram-negative bacteria. Gram-positive bacteria such as Staphylococcus aureus and Streptococcus mutans, which are found in the oral mucosa of denture users, as well as gram-negative bacteria such as Escherichia coli in the digestive tract and bladder mucosa, showed a synergistic relationship, while the antagonistic relationship is shown in gram-positive bacteria such as Lactobacillus spp. in the vulvovaginal environment and Pseudomonas aeruginosa, which are frequently co-isolated from contaminated catheters and chronic lung infections [25],[72],[73],[75].
The formation of mixed species biofilms involving C. albicans and bacteria has been described extensively; however, little has addressed intra-genus interactions between Candida species. Candida albicans has been studied more extensively than any other NAC species, although the role of NAC species has received increasing attention over time by clinicians [25]. Unfortunately, the interactions, except for C. albicans on host responses, are poorly characterized. Abrantes et al. [76] reported that Candida mixed biofilm interactions using an impedance-based biofilm monitoring system showed that the maximum cell adherence index increased over time in most mixed biofilms more than in monocultures, except C. albicans. Cell enhancement can be due to synergistic activity between NAC species that restore matrix structure and function, which allows species deficient in certain carbohydrates to be supplemented by their neighbors with different carbohydrates. When using the conventional crystal violet staining, the most biofilm formation was observed in mixed C. albicans and C. tropicalis biofilms, with the lowest being observed for the C. glabrata/ C. parapsilosis combination [76].
Candida albicans forms antagonistic interactions with NAC species in mixed biofilms, while the combination will benefit NAC over C. albicans since this species produces more significant amounts of the ECM when in a monoculture [77]–[79]. A study by Pathirana et al. [80] showed that, out of five NAC species (C. tropicalis, C. dubliniensis, C. parapsilosis, C. lusitaniae and C. krusei), only C. tropicalis and C. dubliniensis were able to attach to C. albicans hyphae. These two NAC species share a close phylogenetic relationship with C. albicans and the ability to form true hyphae. This mixed biofilm shows a growth advantage for both NAC species and not for C. albicans. Furthermore, Santos et al. [77] showed that, among the NAC species, C. krusei had the highest inhibitory activity against the in vitro biofilm model of C. albicans. These results suggest that there may be nutritional competition between these species, or C. krusei generating signaling molecules that inhibit C. albicans growth. Another study from Barros et al. [81] regarding the combination biofilms of C. albicans with C. krusei indicates a competitive antagonistic interaction between these two species by altering or inhibiting the mechanisms involved in the in vitro adherence and formation of C. albicans biofilms. Candida krusei can inhibit the expression of the Als1, Als3, Hwp1, Bcr1, Efg1 and Tec1 genes of C. albicans during in vitro biofilm production. Meanwhile, biofilms mixed with C. albicans and C. glabrata also showed a decrease in C. albicans biofilm mass; however, there was a slight increase in the expression of Als3, Hwp1, Tec1 and Bcr1 from C. albicans; thus, this study suggests a neutral relationship, or even synergism [81].
Efforts to control the formation of Candida spp. biofilms are still challenging. Biofilms can readily colonize on permanent medical devices used by patients, such as intravascular catheters, urinary catheters or prosthetic heart valves, where prolonged use, especially in critically ill patients, increases the risk of developing candidemia, resulting in sepsis [1],[9],[15]. In patients with CRBSI caused by Candida spp., catheter removal is recommended for decreased mortality [82]–[84]. However, occasionally, catheter removal is impossible in patients such as neonates with extremely low birth weight, patients with surgical catheter implants without other available vascular access or those with severe coagulopathy [83],[85]. The availability of antifungal drug options for treating systemic candidiasis is limited to azoles, echinocandins and polyenes [86]. Moreover, each of these groups has its challenges; where the azole group acts as a fungistatic rather than a fungicidal drug for Candida, there is increased resistance due to general and long-term use, and several Candida species show intrinsic resistance to fluconazole [54],[87]. Echinocandins appear to be the answer for azole-resistant yeasts, but prolonged exposure of azole drugs to Candida isolates has reduced its susceptibility, especially in immunocompromised patients with recurrent candidemia [54]. Amphotericin B is considered the ultimate therapy, although it has infusion-related toxicity as a side effect, especially in conventional formulations [88]. Hence, numerous prevention and therapeutic methods have evolved to replace or combine with systemic antifungal treatment.
Prevention of biofilm formation related to catheter use currently has two methods, i.e., lock therapy and catheter coating [1],[14]. The lock therapy methods inhibit Candida's attachment to the surface, which is the initial stage of biofilm formation. In this approach, prior to contact with the patient, it is possible to inject high concentrations of antimicrobial agents (usually from 100 to 1000 times the minimal inhibitory concentration (MIC)) into the lumen of the catheter and leave them for several hours or days [83],[89]. The advantage of this method is that it prevents systemic toxicity because it only acts in the catheter; yet, this strategy against Candida can fail to eradicate it if biofilms are formed on the extraluminal of the catheter [1],[14].
Based on in vitro and in vivo studies, the most promising antifungal in lock therapy against Candida biofilms are echinocandins and amphotericin B; however, with the azole group, they are less effective [89]–[91]. Echinocandins inhibit the synthesis of β-(1,3)-d-glucan, an essential component of fungal cell walls, with anti-biofilm activity that is effective even at low concentrations approaching the MIC [54]. Caspofungin, micafungin and anidulafungin are echinocandins used in lock therapy [83]. Caspofungin is the class of echinocandin most widely explored as a type of lock therapy owing to its anti-biofilm activity against C. albicans, C. auris, C. lusitaniae, C. glabrata and C. guilliermondii [83],[92]. Interestingly, micafungin can reduce the metabolic activity of the C. albicans biofilm by up to 98% if combined with 20% ethanol and 800 µg/mL doxycycline. In addition, micafungin can effectively eliminate catheter-associated candidemia in male infants if combined with 0.3 mL of 70% ethanol [93].
Furthermore, micafungin and anidulafungin are recommended for C. glabrata since they show much higher activity as compared to liposomal amphotericin B (LAmB)-based in vitro studies [94]. Amphotericin B acts by binding to ergosterol in the fungal cell membrane, which induces the formation of aqueous pores and the sequestration of ergosterol, which triggers oxidative damage. [54],[91]. An in vivo study by Fujimoto and Takemoto [91] showed that using LAmB was more effective in eradicating Candida biofilms in catheters when given a combination of systemic and lock therapies, as compared to micafungin. The LAmB administration from in vitro studies also shows superior activity for C. albicans and C.tropicalis, while, for C. parapsilosis, both micafungin and LAmB are comparable [91].
Methods of coating on the surface of a catheter with biomaterial innovations, such as antifungals, polymers and nanoparticles, have been explored [1],[95]. Caspofungin is the antifungal class of echinocandins, with primary amines often used as coating materials to prevent fungal cell attachment using covalent interactions [96]–[99]. Interestingly, Naderi et al. [100] demonstrated that coating with other echinocandins, such as anidulafungin and micafungin, can also eliminate 106 CFU/cm2 inoculation of C. albicans and prevent biofilm formation and conversion to hyphae. Although surface coating with Food and Drug Administration (FDA) approved antifungal is promising, there is concern about increasing resistance in this way. An alternative was developed by using small molecules and peptides with antifungal and anti-biofilm properties [95]. Filastatin is a small-molecule inhibitor that effectively inhibits the attachment of C. albicans to the surface of medical devices [101]. Biomedical surfaces coated layer-by-layer and combined with the antifungal 14-helical β-peptide structure can efficiently eliminate and inhibit biofilm formation when administered in bulk solution to planktonic populations of C. albicans, or when released slowly from polymer coatings containing embedded β-peptides [102],[103]. Polymer-based catheter coatings also possess antifungal and/or antibiofilm properties, with the advantage of being less susceptible to resistance than small-molecule antifungals, making them an effective alternative in candidemia treatment. Chitosan is a bioactive polymer from naturally derived polysaccharides; it is widely incorporated into hydrogels and used as a coating to prevent Candida attachment and the formation of biofilms [104]. Another type of coating material that has developed rapidly in recent years is chitosan-coated polymeric silver and gold nanoparticles, which have anti-biofilm effects and may increase the bioavailability of antifungal drugs. In addition, they have the advantage of being able to penetrate EPSs and target fungal cells so that they can be used as Candida biofilm therapy [105],[106].
Another alternative developed to treat Candida biofilms is natural peptide products from plant extracts, microbes, insects or other sources [107],[108]. Antifungal peptides consist of various classes, with the most widely studied classes related to fungal biofilms being defensins, cathelicidins and histatins. These compounds act by preventing the formation of biofilms and eradicating preformed ones through various mechanisms, including disrupting fungal membrane integrity, inhibiting the adhesion of planktonic fungal cells to the surface, disrupting gene regulation and inducing the production of reactive oxygen species [102]. While this alternative is promising, the toxicity of these compounds has not been established, and the doses tolerated in patients need to be studied further [109].
Recently, an alternative approach using photodynamic inactivation (PDI) to eliminate Candida biofilms has been developed with a different class of non-toxic dyes called photosensitizers (PSs) [1],[110],[111]. This technique uses visible light at the appropriate wavelength and a PS to induce cell damage and death through apoptosis or necrosis in target cells. When the PS is activated by light, it will react to oxygen and make the microorganisms produce reactive oxygen species, causing damage to the DNA and increased cell membrane permeability [111]–[113]. Various classes of PSs have been reported for PDI in C. albicans, including curcumin, methylene blue (the most commonly used), porphyrin and toluidine blue [110],[111]. This approach is effective against drug-resistant strains and does not show the potential to develop drug resistance [110],[114]. In addition, it has been proven that combining PDI with antifungal agents can increase the planktonic killing power of C. albicans [110],[115],[116]. All of the strategies for the prevention and therapy of catheter-associated Candida biofilms that are suggested to replace or be combined with systemic antifungal treatment are summarized in Table 2.
Methods | Recommendation and notes | References |
Lock therapy | Echinocandins (caspofungin, micafungin and anidulafungin) and amphotericin B were used as lock solutions (intraluminal) for both prevention and treatment of catheter-related infections. | [85]–[87] |
Catheter coating | Caspofungin is the most commonly used in direct surface coating for prevention. Small molecule (filastatin), polymers (chitosan) and nanoparticles (silver or gold) demonstrated antibiofilm activity for alternative coating. |
[92]–[95],[97],[100]–[102] |
Natural peptide products | Defensins, cathelicidins and histatin are the alternative peptides from plant extracts, microbes, insects or other sources that show antifungal activity to inhibit Candida biofilm. | [98] |
Photodynamic inactivation | Methylene blue is the most commonly used PS to inhibit the viability of the biofilm produced by Candida. | [106],[107] |
Candida is a common source of nosocomial infection due to its ability to form biofilms on medical devices, especially CVCs in ICU patients. Candida albicans and C. parapsilosis are species that are often isolated in association with biofilms due to their ability in morphogenesis to facilitate the occurrence of persistent infections associated with catheter use. This review also highlights the complexity of Candida species biofilm formation and the different characteristics between C. albicans and NAC species, such as the morphology, unique ECM composition and antifungal resistance. Therefore, recent management and prevention of Candida biofilm, including lock therapy, catheter coating methods, natural peptides and PDI, can be effective despite the limited antifungal armamentarium and increasing drug resistance.
[1] | Bayes T (1763) An Essay towards Solving a Problem in the Doctrine of Chances by the Late Rev. Mr. Bayes, F. R. S. Communicated by Mr. Price, in a Letter to John Canton, AMFRS. Philos T 53: 370–418. |
[2] |
Boamah N (2015) Robustness of the Carhart four-factor and the Fama-French three-factor models on the South African stock market. Rev Account Financ 14: 413–430. https://doi.org/10.1108/RAF-01-2015-0009 doi: 10.1108/RAF-01-2015-0009
![]() |
[3] | Bouzinne YD, Muller-Bosse S, Steen H, et al. (2019) The five-factor asset pricing model - A theoretical review and assessment. Manage Stud 9: 2–7. https://managementstudies.org/ms/article/view/8 |
[4] |
Butt AB, Kolari JW, Sadaqat M (2021) Revisiting momentum profits in emerging markets. Pacific-Basin Financ J 65: 1–20. https://doi.org/10.1016/j.pacfin.2020.101486 doi: 10.1016/j.pacfin.2020.101486
![]() |
[5] |
Charteris A, Rwishema M, Chidede T (2018) Asset Pricing and Momentum: A South African Perspective. J Afr Bus 19: 62–85. http://dx.doi.org/10.1080/15228916.2017.1343033 doi: 10.1080/15228916.2017.1343033
![]() |
[6] | Chen L, Novy-Marx R, Zhang L (2011) An alternative three-factor model. Soc Sci Res Network Work. Paper No: 1418117. https://dx.doi.org/10.2139/ssrn.1418117 |
[7] |
Cox S, Britten J (2019) The Fama-French five-factor model: Evidence from the Johannesburg Stock Exchange. Investment Anal J 48: 240–261. https://doi.org/10.1080/10293523.2019.1647982 doi: 10.1080/10293523.2019.1647982
![]() |
[8] |
Dutta A (2019) Does the Five-Factor Asset Pricing Model Have Sufficient Power? Global Bus Rev 20: 684–691. http://dx.doi.org/10.1177/0972150919837060 doi: 10.1177/0972150919837060
![]() |
[9] |
Fama EF, Macbeth JD (1973) Risk, return, and equilibrium: empirical tests. J Polit Econ 81: 607–636. http://dx.doi.org/10.1086/260061 doi: 10.1086/260061
![]() |
[10] |
Fama E, French K (1996) Multifactor explanations of asset pricing anomalies. J Financ 51: 55–84. https://doi.org/10.1111/j.1540-6261.1996.tb05202.x doi: 10.1111/j.1540-6261.1996.tb05202.x
![]() |
[11] |
Fama E, French K (2015) A five-factor asset pricing model. J Financ Econ 116: 1–22. https://doi.org/10.1016/j.jfineco.2014.10.010 doi: 10.1016/j.jfineco.2014.10.010
![]() |
[12] |
Foye J (2018) A comprehensive test of the Fama-French five-factor model in emerging markets. Emerg Mark Rev 37: 199–222. https://doi.org/10.1016/j.ememar.2018.09.002 doi: 10.1016/j.ememar.2018.09.002
![]() |
[13] |
Gelfand AE, Ghosh SK (1998) Model choice: A minimum posterior predictive loss approach. Biometrika 85: 1–11. https://doi.org/10.1093/biomet/85.1.1 doi: 10.1093/biomet/85.1.1
![]() |
[14] |
González-Sánchez M (2021) Term Structure of Risk Factor Premiums Used for Pricing Asset: Emerging vs. Developed Markets. Emerg Mark Financ Trade 58: 1399–1358. https://doi.org/10.1080/1540496X.2021.1873128 doi: 10.1080/1540496X.2021.1873128
![]() |
[15] |
Gow ID, Ormazabal G, Taylor DJ (2010) Correcting for Cross-Sectional and Time-Series Dependence. Account Rev 85: 483–512. https://doi.org/10.2308/accr.2010.85.2.483 doi: 10.2308/accr.2010.85.2.483
![]() |
[16] | Hasnawati S (2020) Size, Return and Public Company's Performance: A Study Small and Large Companies on IDX During 3 Economic-Periods. Acad Account Financ Stud J 24: 1–7. http://repository.lppm.unila.ac.id/id/eprint/19563 |
[17] |
Hudson RS, Gregoriou A (2015) Calculating and comparing security returns is harder than you think: A comparison between logarithmic and simple returns. Int Rev Financ Anal 38: 151–162. http://dx.doi.org/10.1016/j.irfa.2014.10.008 doi: 10.1016/j.irfa.2014.10.008
![]() |
[18] |
Jegadeesh N, Titman S (1993) Returns to buying winners and selling losers: Implications for stock market efficiency. J Financ 48: 65–91. https://doi.org/10.1111/j.1540-6261.1993.tb04702.x doi: 10.1111/j.1540-6261.1993.tb04702.x
![]() |
[19] |
Jensen MJ, Maheu JM (2018) Risk, Return and Volatility Feedback: A Bayesian Nonparametric Analysis. J Risk Financ Manage11: 1–29. https://doi.org/10.3390/jrfm11030052 doi: 10.3390/jrfm11030052
![]() |
[20] |
Kalli M, Griffin J, Walker S (2011) Slice sampling mixture models. Stat Comput 21: 93–105. https://doi.org/10.1007/s11222-009-9150-y doi: 10.1007/s11222-009-9150-y
![]() |
[21] |
Karabatsos G (2017) A menu-driven software package of Bayesian nonparametric (and parametric) mixed models for regression analysis and density estimation. Behav Res Methods 49: 335–362. https://doi.org/10.3758/s13428-016-0711-7 doi: 10.3758/s13428-016-0711-7
![]() |
[22] | MacKenzie D, Nichols J, Royle J, et al. (2018) Fundamental Principals of Statistical Inference. http://dx.doi.org/10.1016/B978-0-12-407197-1.00004-1 |
[23] |
Markowitz H (1952) Portfolio selection. J Finance 7: 77–91. https://doi.org/10.1111/j.1540-6261.1952.tb01525.x doi: 10.1111/j.1540-6261.1952.tb01525.x
![]() |
[24] |
Molele MH, Mukuddem-Petersen J (2020) Emerging market currency risk exposure: evidence from South Africa. J Risk Financ 21: 159–179. https://doi.org/10.1108/JRF-07-2019-0123 doi: 10.1108/JRF-07-2019-0123
![]() |
[25] | Mpoha S, Bonga-Bonga L (2020) Assessing the extent of exchange rate risk pricing in equity markets: emerging versus developed economies. Munich Personal RePEc Archive (MPRA) Paper 99597. University of Johannesburg. South Africa. https://mpra.ub.uni-muenchen.de/99597/ |
[26] | National Treasury (2021) Economic Overview. In: Chapter 2. Budget Review. Republic of South Africa. Available from: http://www.treasury.gov.za/documents/National%20Budget/2021/review/Chapter%202.pdf |
[27] | Peerbhai F, Strydom BS (2018) Testing the International CAPM's relevance for South Africa. J Contemp Manage 15: 525–550. https://journals.co.za/doi/pdf/10.10520/EJC-154ac329e0 |
[28] |
Reisinger A, van Heerden J (2014) Is liquidity a pricing factor on the JSE? J Stud Econ Econometrics 38: 17–34. http://dx.doi.org/10.1080/10800379.2014.12097261 doi: 10.1080/10800379.2014.12097261
![]() |
[29] |
Sahu SK, Gelfand AE, Holland DM (2006) Spatio-Temporal Modeling of Fine Particulate Matter. J Agric Biol Environ Stat 11: 61–86. http://dx.doi.org/10.1198/108571106X95746 doi: 10.1198/108571106X95746
![]() |
[30] |
Sharpe WF (1964) Capital asset prices: A theory of market equilibrium under conditions of risk. J Financ 19: 425–442. https://doi.org/10.1111/j.1540-6261.1964.tb02865.x doi: 10.1111/j.1540-6261.1964.tb02865.x
![]() |
[31] |
Steyn JP, Theart L (2019) Are South African equity investors rewarded for taking on more risk? J Econ Financ Sci 12: 1–10. https://doi.org/10.4102/jef.v12i1.448 doi: 10.4102/jef.v12i1.448
![]() |
[32] | Zaiontz C (2022) Real Statistics Using Excel. Available from: https://www.real-statistics.com/. |
1. | Jamile de Paiva Macedo, Vanessa Cordeiro Dias, Antifungal resistance: why are we losing this battle?, 2024, 19, 1746-0913, 1027, 10.1080/17460913.2024.2342150 | |
2. | Jingwen Zhang, Guoqiang Zhang, JiaJia Wang, Yun Xiao, Xinxin Lu, Xunhong Lan, Yan Zhang, Zhang Dai, Establishment and Validation of a Nomogram Clinical Prediction Model for Nosocomial Candidemia: An 18-Year Retrospective Analysis, 2024, Volume 17, 1178-6973, 4455, 10.2147/IDR.S480028 | |
3. | Maria Baltogianni, Vasileios Giapros, Niki Dermitzaki, Recent Challenges in Diagnosis and Treatment of Invasive Candidiasis in Neonates, 2024, 11, 2227-9067, 1207, 10.3390/children11101207 | |
4. | Shamshe Shaik, Jin-Hyung Lee, Yong-Guy Kim, Jintae Lee, Antifungal, anti-biofilm, and anti-hyphal properties of N-substituted phthalimide derivatives against Candida species, 2024, 14, 2235-2988, 10.3389/fcimb.2024.1414618 | |
5. | Cornelia Lass-Flörl, Souha S. Kanj, Nelesh P. Govender, George R. Thompson, Luis Ostrosky- Zeichner, Miriam Alisa Govrins, Invasive candidiasis, 2024, 10, 2056-676X, 10.1038/s41572-024-00503-3 | |
6. | A.L. Francis, S. Karthick Raja Namasivayam, K. Samrat, Potential of silver nanoparticles synthesized from Justicia adhatoda metabolites for inhibiting biofilm on urinary catheters, 2024, 196, 08824010, 106957, 10.1016/j.micpath.2024.106957 | |
7. | Yuxin Song, Junyao Wang, Xi Liu, Shengwei Yu, Xing Tang, Huaxin Tan, LC-AMP-F1 Derived from the Venom of the Wolf Spider Lycosa coelestis, Exhibits Antimicrobial and Antibiofilm Activities, 2024, 16, 1999-4923, 129, 10.3390/pharmaceutics16010129 | |
8. | Mba Ifeanyi Elibe, Nweze Emeka Innocent, 2024, Chapter 9, 978-981-97-4908-9, 239, 10.1007/978-981-97-4909-6_9 | |
9. | Niki Dermitzaki, Foteini Balomenou, Dimitra Gialamprinou, Vasileios Giapros, Dimitrios Rallis, Maria Baltogianni, Perspectives on the Use of Echinocandins in the Neonatal Intensive Care Unit, 2024, 13, 2079-6382, 1209, 10.3390/antibiotics13121209 | |
10. | Gabriel Grube dos Santos, Amanda Cristina Zangirolami, Maria Luiza Ferreira Vicente, Vanderlei Salvador Bagnato, Kate Cristina Blanco, Photodynamic therapy as a potential approach for preventing fungal spread associated with the use of endotracheal tubes, 2024, 0031-8655, 10.1111/php.14054 | |
11. | Gabriel Grube Dos Santos, Kate Cristina Blanco, Vanderlei Salvador Bagnato, 2024, Investigation of Photobleaching Dynamics of Functionalized Curcumin in PVC for Antimicrobial Photodynamic Applications, 979-8-3503-8817-6, 1, 10.1109/SBFotonIOPC62248.2024.10813508 | |
12. | Olga I. Guliy, Stella S. Evstigneeva, Bacterial Communities and Their Role in Bacterial Infections, 2024, 16, 1945-0494, 10.31083/j.fbe1604036 | |
13. | Farid M. Sroor, Ahmed Younis, Mohamed Abdelraof, Ismail A. Abdelhamid, Synthesis, molecular docking and anti-biofilm activity of novel benzo[4,5]imidazo[2,1-a]quinazoline, 4H-chromene, and acridine derivatives as potent anti-candida agents, 2025, 00222860, 141520, 10.1016/j.molstruc.2025.141520 | |
14. | Niki Dermitzaki, Natalia Atzemoglou, Vasileios Giapros, Maria Baltogianni, Dimitrios Rallis, Theodoros Gouvias, Anastasios Serbis, Aikaterini Drougia, Elimination of Candida Sepsis and Reducing Several Morbidities in a Tertiary NICU in Greece After Changing Antibiotic, Ventilation, and Nutrition Protocols, 2025, 14, 2079-6382, 159, 10.3390/antibiotics14020159 | |
15. | Antonia Thassya Lucas dos Santos, Maria Audilene de Freitas, Maria Lucilene Queiroz da Silva, Francildo dos Santos Silva, Andressa Guilhermino dos Santos, Aparecida Vitória Silva Menêses, Naiza Saraiva Farias, Joara Nályda Pereira Carneiro, Victor Juno Alencar Fonseca, Hélcio Silva dos Santos, Francisco Rogenio da Silva Mendes, Jacilene Silva, Márcia Machado Marinho, Emmanuel Silva Marinho, Henrique Douglas Melo Coutinho, Maria Flaviana Bezerra Morais-Braga, In silico activity and effect of synthetic chalcones on Candida albicans and Candida tropicalis biofilms, 2025, 03009084, 10.1016/j.biochi.2025.03.004 | |
16. | Maria Inês Pacheco, Joana Carvalho-Pereira, Augusto Costa-Barbosa, Fabiana Marques, Pedro M. Durães, M. Sameiro T. Gonçalves, Maria João Sousa, Paula Sampaio, Benzo[a]phenoxazine derivative C34 efficacy against fluconazole-resistant Candida spp., 2025, 203, 08824010, 107498, 10.1016/j.micpath.2025.107498 | |
17. | Meiliyana Wijaya, Sem Samuel Surja, Hanna Yolanda, Orlin Clarista, Freggy Spicano Joprang, Maria Mardalena Martini Kaisar, Challenges of diagnosis of hidden fungal infections as sequelae in post-coronavirus disease patients, 2025, 2770-3150, 10.1097/MRM.0000000000000435 | |
18. | Andres Ceballos-Garzon, Julien Lebrat, Marion Holzapfel, Diego F. Josa, Jeremy Welsch, Derry Mercer, Andreas H. Groll, Antibiofilm activity of manogepix, ibrexafungerp, amphotericin B, rezafungin, and caspofungin against Candida spp. biofilms of reference and clinical strains , 2025, 0066-4804, 10.1128/aac.00137-25 |
Gene name | Description | Stage |
Als | Consists of eight large cell surface glycoproteins: Als1–7,9; with Als 1, Als3 and Als5 as the most involved in the attachment process | Attachment |
Hwp | Hwp1, Hwp2 and Rbt1 (repressed by Tup1) belong to this gene family, which is required for adhesion to host cell surface proteins and cell aggregationHwp1 is also required for hyphal formation | Attachment and transitional |
Bcr1 | Important in the adhesion process of yeast cells to the surface, and also acts as a trancription factor for upregulation in Hwp1 expression | Attachment and transitional |
Efg1 | ||
Brg1 | Transcription factor to promote hyphal formation | Transitional |
Rob1 | ||
Tec1 | ||
Ndt80 | ||
Zap1 | Negative regulator of ECM production | Maturation |
Rlm1 | Positive regulator of ECM production | |
Nrg1 | Overexpression leads to increased dispersion cells | Dispersion |
Pse1 | ||
Ume6 | Overexpression leads to reduced dispersion cells | Dispersion |
Methods | Recommendation and notes | References |
Lock therapy | Echinocandins (caspofungin, micafungin and anidulafungin) and amphotericin B were used as lock solutions (intraluminal) for both prevention and treatment of catheter-related infections. | [85]–[87] |
Catheter coating | Caspofungin is the most commonly used in direct surface coating for prevention. Small molecule (filastatin), polymers (chitosan) and nanoparticles (silver or gold) demonstrated antibiofilm activity for alternative coating. |
[92]–[95],[97],[100]–[102] |
Natural peptide products | Defensins, cathelicidins and histatin are the alternative peptides from plant extracts, microbes, insects or other sources that show antifungal activity to inhibit Candida biofilm. | [98] |
Photodynamic inactivation | Methylene blue is the most commonly used PS to inhibit the viability of the biofilm produced by Candida. | [106],[107] |
Gene name | Description | Stage |
Als | Consists of eight large cell surface glycoproteins: Als1–7,9; with Als 1, Als3 and Als5 as the most involved in the attachment process | Attachment |
Hwp | Hwp1, Hwp2 and Rbt1 (repressed by Tup1) belong to this gene family, which is required for adhesion to host cell surface proteins and cell aggregationHwp1 is also required for hyphal formation | Attachment and transitional |
Bcr1 | Important in the adhesion process of yeast cells to the surface, and also acts as a trancription factor for upregulation in Hwp1 expression | Attachment and transitional |
Efg1 | ||
Brg1 | Transcription factor to promote hyphal formation | Transitional |
Rob1 | ||
Tec1 | ||
Ndt80 | ||
Zap1 | Negative regulator of ECM production | Maturation |
Rlm1 | Positive regulator of ECM production | |
Nrg1 | Overexpression leads to increased dispersion cells | Dispersion |
Pse1 | ||
Ume6 | Overexpression leads to reduced dispersion cells | Dispersion |
Methods | Recommendation and notes | References |
Lock therapy | Echinocandins (caspofungin, micafungin and anidulafungin) and amphotericin B were used as lock solutions (intraluminal) for both prevention and treatment of catheter-related infections. | [85]–[87] |
Catheter coating | Caspofungin is the most commonly used in direct surface coating for prevention. Small molecule (filastatin), polymers (chitosan) and nanoparticles (silver or gold) demonstrated antibiofilm activity for alternative coating. |
[92]–[95],[97],[100]–[102] |
Natural peptide products | Defensins, cathelicidins and histatin are the alternative peptides from plant extracts, microbes, insects or other sources that show antifungal activity to inhibit Candida biofilm. | [98] |
Photodynamic inactivation | Methylene blue is the most commonly used PS to inhibit the viability of the biofilm produced by Candida. | [106],[107] |