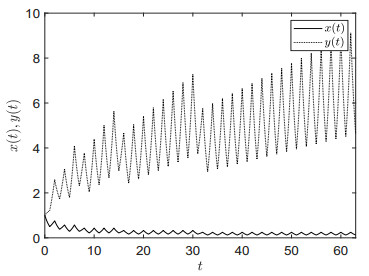
One of the most famous equations that are widely used in various branches of physics, mathematics, financial markets, etc. is the Langevin equation. In this work, we investigate the existence of the solution for two generalized fractional hybrid Langevin equations under different boundary conditions. For this purpose, the problem of the existence of a solution will become the problem of finding a fixed point for an operator defined in the Banach space. To achieve the result, one of the recent fixed point techniques, namely the α-ψ-contraction technique, will be used. We provide sufficient conditions to use this type of contraction in our main theorems. In the calculations of the auxiliary lemmas that we present, the Mittag-Leffler function plays a fundamental role. The fractional derivative operators used are of the Caputo type. Two examples are provided to demonstrate the validity of the obtained theorems. Also, some figures and a table are presented to illustrate the results.
Citation: Zohreh Heydarpour, Maryam Naderi Parizi, Rahimeh Ghorbnian, Mehran Ghaderi, Shahram Rezapour, Amir Mosavi. A study on a special case of the Sturm-Liouville equation using the Mittag-Leffler function and a new type of contraction[J]. AIMS Mathematics, 2022, 7(10): 18253-18279. doi: 10.3934/math.20221004
[1] | Yanchao He, Yuzhen Bai . Finite-time stability and applications of positive switched linear delayed impulsive systems. Mathematical Modelling and Control, 2024, 4(2): 178-194. doi: 10.3934/mmc.2024016 |
[2] | Hongyu Ma, Dadong Tian, Mei Li, Chao Zhang . Reachable set estimation for 2-D switched nonlinear positive systems with impulsive effects and bounded disturbances described by the Roesser model. Mathematical Modelling and Control, 2024, 4(2): 152-162. doi: 10.3934/mmc.2024014 |
[3] | Hongwei Zheng, Yujuan Tian . Exponential stability of time-delay systems with highly nonlinear impulses involving delays. Mathematical Modelling and Control, 2025, 5(1): 103-120. doi: 10.3934/mmc.2025008 |
[4] | Yunsi Yang, Jun-e Feng, Lei Jia . Recent advances of finite-field networks. Mathematical Modelling and Control, 2023, 3(3): 244-255. doi: 10.3934/mmc.2023021 |
[5] | Wen Zhang, Jinjun Fan, Yuanyuan Peng . On the discontinuous dynamics of a class of 2-DOF frictional vibration systems with asymmetric elastic constraints. Mathematical Modelling and Control, 2023, 3(4): 278-305. doi: 10.3934/mmc.2023024 |
[6] | Bangxin Jiang, Yijun Lou, Jianquan Lu . Input-to-state stability of delayed systems with bounded-delay impulses. Mathematical Modelling and Control, 2022, 2(2): 44-54. doi: 10.3934/mmc.2022006 |
[7] | Lusong Ding, Weiwei Sun . Neuro-adaptive finite-time control of fractional-order nonlinear systems with multiple objective constraints. Mathematical Modelling and Control, 2023, 3(4): 355-369. doi: 10.3934/mmc.2023029 |
[8] | Yulin Guan, Xue Zhang . Dynamics of a coupled epileptic network with time delay. Mathematical Modelling and Control, 2022, 2(1): 13-23. doi: 10.3934/mmc.2022003 |
[9] | Shipeng Li . Impulsive control for stationary oscillation of nonlinear delay systems and applications. Mathematical Modelling and Control, 2023, 3(4): 267-277. doi: 10.3934/mmc.2023023 |
[10] | Gani Stamov, Ekaterina Gospodinova, Ivanka Stamova . Practical exponential stability with respect to h−manifolds of discontinuous delayed Cohen–Grossberg neural networks with variable impulsive perturbations. Mathematical Modelling and Control, 2021, 1(1): 26-34. doi: 10.3934/mmc.2021003 |
One of the most famous equations that are widely used in various branches of physics, mathematics, financial markets, etc. is the Langevin equation. In this work, we investigate the existence of the solution for two generalized fractional hybrid Langevin equations under different boundary conditions. For this purpose, the problem of the existence of a solution will become the problem of finding a fixed point for an operator defined in the Banach space. To achieve the result, one of the recent fixed point techniques, namely the α-ψ-contraction technique, will be used. We provide sufficient conditions to use this type of contraction in our main theorems. In the calculations of the auxiliary lemmas that we present, the Mittag-Leffler function plays a fundamental role. The fractional derivative operators used are of the Caputo type. Two examples are provided to demonstrate the validity of the obtained theorems. Also, some figures and a table are presented to illustrate the results.
Switched cascade nonlinear systems (SCNSs) combine both features of switched systems [1,2,3,4,5] and cascade systems [6,7,8,9] and are characterized by complex dynamics and broad applications [10,11,12]. On this ground, a great number of researchers have intensively investigated dynamics, especially stability and control issues, of SCNSs, see [13,14,15,16] and the references therein.
Consider the following SCNS with delays:
˙x(t)=hσ(t)(t,xt,d(t)), | (1.1a) |
˙y(t)=fσ(t)(t,yt,d(t))+gσ(t)(t,xt,d(t)), | (1.1b) |
where d(t) is delay, σ(t) is a switching signal. System (1.1a) and
˙y(t)=fσ(t)(t,yt,d(t)), | (1.2) |
are called separate systems 1 and 2 of cascade system (1.1), respectively, and the term gσ(t) is the coupling term. Clearly, evolution of (1.1a) is completely determined by (1.1a) itself and it affects that of system (1.1b) via gσ(t).
Lyapunov theory is a powerful and popular tool to study stability of cascade systems [17,18,19]. Since cascade systems have particular structure, a widely employed method is composite Lyapunov function (functional) [20] which is based on separate Lyapunov function (functional) constructed for each separate system. This method uses an intuitive idea: The property of a cascade system is related to all separate systems and the coupling terms among them.
Note that (1.1b) can be viewed as perturbed system of (1.2) with perturbation gσ(t). It would be desirable that one can draw a stability conclusion for system (1.1) from stability properties of systems (1.1a) and (1.2) and property of perturbation gσ(t). Actually, several papers have been reported on stability of delayed SCNSs using the intuitive point of view. Suppose always that coupling term gσ(t) has a linear growth bound [21]. With the assumption that system (1.2) is exponentially stable, it was revealed that system (1.1) is exponentially stable if and only if so is system (1.1a), and that it is asymptotically stable if so is system (1.1a) [14,16]. These results essentially rely on the following key observation: For a nominal delayed switched nonlinear system being exponentially stable, trajectories of the corresponding perturbed system exponentially (asymptotically) decay to origin if the perturbation can be upper bounded by a function exponentially (asymptotically) converging to zero. Note that many systems are asymptotically stable but not exponentially stable since asymptotic stability is weaker than exponential one. Therefore, it is more significant to study the situation where the nominal systems are asymptotically stable. Recently, it was proposed in Ref. [22] that for a nominal discrete-time switched system being asymptotically stable, trajectories of the corresponding perturbed system asymptotically converge to zero if the perturbation exponentially approaches zero. Based on this fact and with assumption that separate system 2 is asymptotically stable, it was shown that a discrete-time SCNS is asymptotically stable if separate system 1 is exponentially stable [22].
Our motivations are as follows: (ⅰ) The key assumption in Ref. [14] is that separate system 2 is exponentially stable. The stability property keeps unknown in the situation where separate system 2 is asymptotically stable and will be investigated in the present paper. (ⅱ) Explore the robust convergence of an asymptotically stable nominal system with perturbations. Since asymptotic stability is much weaker than exponential one, the issue discussed here is of more importance than that in [14]. (ⅲ) Provide a counterpart of Ref. [22] for continuous-time SCNSs, which is more challenging.
The main contribution lies in three aspects: (ⅰ) The concept of uniform switching signals is proposed, which makes main results in the present paper applicable to many classes of switched systems. (ⅱ) It is shown that, with the assumption that the nominal delayed switched nonlinear system is asymptotically stable, trajectories of the perturbed system asymptotically approach zero if the perturbation can be upper bounded by an exponentially decaying function. (ⅲ) Some sufficient asymptotic stability conditions are presented for continuous-time delayed SCNSs. These results can be applied to stability analysis and controller design for SCNSs in a decomposition way.
The rest is organized as follows. Preliminaries are presented in Section 2. Convergence property of delayed switched nonlinear systems subject to perturbations is discussed in Subsection 3.1, and stability conditions of SCNSs are proposed in Subsection 3.2. A numerical example is provided in Section 4. Finally, Section 5 concludes this paper.
Notation. Rn×m is the set of n×m-dimensional real matrices and Rn=Rn×1. R0,+=[0,∞) and R+=(0,∞). N0={0,1,2,…} and N={1,2,…}. AT is the transpose of matrix A. Given vectors x,y, col(x,y)=[xTyT]T. For a fixed real number a, ⌈a⌉ is the minimum integer greater than or equal to a and |a| is its absolute value. ‖x‖∞=max{|x1|,…,|xn|} is the l∞ norm of x∈Rn and is denoted by ‖x‖. For any continuous function x(s) on [−d,a) with scalars a>0, d>0 and any t∈[0,a), xt denotes a continuous function on [t−d,t] defined by xt(θ)= x(t+θ) for each θ∈[−d,0]. ‖xt‖= supt−d≤s≤t{‖x(s)‖}. C([a,b],Rn) is the set of continuous functions from interval [a,b] to Rn, and Cϑ([a,b],Rn)={x∈C([a,b],Rn):‖x‖<ϑ} with ϑ>0. Let κ∈(0,∞]. A continuous function α:[0,κ)→[0,∞) belongs to class K if it is strictly increasing and α(0)=0, and a continuous function β:[0,κ)×[0,∞)→[0,∞) belongs to class KL, if for each fixed s, β(r,s) belongs to class K with respect to r, and for each fixed r, β(r,s) is decreasing with respect to s and β(r,s)→0 as s→∞ [21]. Throughout this paper, the dimensions of matrices and vectors will not be explicitly mentioned if clear from context.
Consider the following switched nonlinear system:
˙x(t)=fσ(t)(t,xt,d(t)),t≥t0,x(t)=φ(t),t∈[t0−d,t0], | (2.1) |
where t0≥0, x(t)∈Rn is the state, σ:[t0,∞)→{1,…,m} is a switching signal with m being the number of subsystems and is piecewise constant and continuous from the right. It is assumed in the sequel that σ is with switching sequence {ti}∞i=0. d(t)=col(d1(t),…,dι(t))∈Dι≜[d11,d12]×⋯×[dι1,dι2], where di2≥di1≥0,∀i∈{1,…,ι}, and di(t)∈[di1,di2] is piecewise continuous [23]. d=maxi∈{1,…,ι}{di2}. For each l∈{1,…,m}, fl maps [t0,∞)×C([−d,0],Rn)×Dι into Rn. φ∈C([t0−d,t0],Rn) is an initial vector-valued function.
Remark 2.1. By definition, xt has implicitly included the information of d(t) since xt(θ)= x(t+θ),∀θ∈[−d,0]. Thus, the parameter d(t) in system (2.1) is somewhat redundant in some sense. However, due to the fact that several vectors of delays will be introduced in Subsection 3.2, we prefer to write d(t) explicitly in order to distinguish them. The vector d(t) can describe multiple delays including constant, time-varying, distributed, and interval ones [23].
The following assumption is made for system (2.1).
Assumption 2.1. fl(⋅,0,⋅)=0. fl is continuous on [t0,∞)×C([−d,0],Rn)×Dι and is (locally) Lipschitz in the second argument, uniformly in [t0,∞)×Dι, that is, there exist positive scalars L,ϑ such that
‖fl(⋅,x,⋅)−fl(⋅,y,⋅)‖≤L‖x−y‖,∀x,y∈Cϑ([−d,0],Rn). | (2.2) |
Note that fl is globally Lipschitz if ϑ=∞.
There are many kinds of switching signals in literature which play a fundamental role on system dynamics. To motivate our new concept, let us recall some kinds of familiar switching signals in literature.
1. Periodically switching signals: There exists a scalar κ>0 such that σ(t+κ)=σ(t),∀t≥t0, which clearly implies that σ(t+κ)=σ(t),∀t≥t0+c with c>0.
2. Switching signals having property of finite discontinuities on finite interval: σ has finite discontinuities on any finite interval contained in [t0,∞). Clearly, σ also has finite discontinuities on any finite interval contained in [t0+c,∞) with c>0.
Now introduce the concept of uniform switching signal which includes most switching signals frequently encountered in literature.
Definition 2.1. (Uniform switching signals) A switching property P is said to uniform (with respect to t) if P is valid on [t0+c,∞) for any c>0. A switching signal σ satisfying a uniform property P is said to be a uniform one (with P). The set S(P)={σ:σ has uniform property P} is said to be uniform with P.
One can easily check the following properties are uniform. (ⅰ). Property satisfying average dwell time constraint: There exist positive numbers N0 and τa such that Nσ(T,t)≤N0+T−tτa with Nσ(T,t) being the switching number of σ on the open interval (t,T),∀T>t≥t0 [24]. (ⅱ). Property satisfying dwell time constraint (which is actually a special case of (ⅰ) with N0=1 [24,page 58]). (ⅲ). Fast switching property [25,26]. (ⅳ). Homogeneous Markov switching property [27]. (ⅴ). Property satisfying mode-dependent average dwell time constraint [28].
In the sequel, it is always assumed that the underlying set S is uniform with certain given P. Since we do not discuss any specific P, P will not be mentioned explicitly. Moreover, when speaking of "a switched system has some dynamic property", we mean that the property holds for any σ∈S.
It is worth noting that there may exist some relationship between different uniform P's and that for a given switched system with specified subsystems, its dynamic property may vary with P. If P1 is "arbitrary switching" and P2 has a dwell time τd, then it may occur that a system is asymptotically stable over S(P2) but diverges over S(P1). Conversely, if a system is asymptotically stable over S(P1) then it is also asymptotically stable over S(P2) since S(P2)⊆S(P1).
Though most switching signals possess uniformity, there do exist exceptions one of which is the so-called Zeno behavior admitting an infinite number of switches in a finite interval [29].
To make notation concise, from now on we denote the solution to (2.1) by x(t;φ) rather than x(t;t0,φ) with starting time t0 and initial function φ defined on [t0−d,t0], since t0 is indicated by φ. xc is the solution to (2.1) on interval [c−d,c] for c≥t0. This convention will be applied to systems (3.1) and (3.40) without further statement.
Definition 2.2. ([30]) Given a set of switching signals S. System (2.1) is locally uniformly exponentially stable (LUES) if there exist positive scalars α,γ,δ satisfying ‖x(t;φ)‖≤αexp(−γ(t−t0))‖φ‖,∀t0≥0,t≥t0,‖φ‖≤δ,d(t)∈Dι,σ∈S; if δ can be arbitrarily large, then it is globally uniformly exponentially stable (GUES). (2.1) is locally uniformly asymptotically stable (LUAS) if there exists a function β of class KL and a scalar δ such that ‖x(t;φ)‖≤β(‖φ‖,t−t0),∀t0≥0,t≥t0,‖φ‖≤δ,d(t)∈Dι,σ∈S; if δ can be arbitrarily large, then it is globally uniformly asymptotically stable (GUAS). The scalar δ is called the radius of attraction if (2.1) is LUES or LUAS.
Now introduce the following lemma:
Lemma 2.1. Let (2.1) be GUAS, that is, there exists a function β∈KL satisfying ‖x(t;φ)‖≤β(‖φ‖,t−t0),∀t≥t0. Fix positive scalars a,b,ν satisfying a≤b. Then there exists a scalar τ>0 such that β(‖φ‖,t−t0)≤ν‖φ‖ and ‖x(t;φ)‖≤ν‖φ‖, ∀t≥t0+τ,a≤‖φ‖≤b.
Proof. Fix a,b,ν. Since (2.1) is GUAS, there exists β∈KL such that ‖x(t;φ)‖≤β(‖φ‖,t−t0). Suppose by contradiction that for any τ>0, there exists a pair (φ,c) with a≤‖φ‖≤b and c≥τ such that β(‖φ‖,c)>ν‖φ‖. Take an increasing positive sequence {τi}∞i=1 with τ1≥t0,limi→∞τi=∞, and there exists a sequence of pairs {(φi,ci)}∞i=1 satisfying a≤‖φi‖≤b,τi≤ci, and β(‖φi‖,ci)>ν‖φ‖. ‖φi‖≤b means limi→∞β(b,ci)≥limi→∞β(‖φi‖,ci)≥ν‖φ‖≥νa>0, contradicting the fact limi→∞β(b,ci)=0. Therefore, there necessarily exists τ>0 satisfying β(‖φ‖,t−t0)≤ν‖φ‖,∀t≥t0+τ,a≤‖φ‖≤b. Since ‖x(t;φ)‖≤β(‖φ‖,t−t0), it is clear that ‖x(t;φ)‖≤ν‖φ‖,∀t≥t0+τ,a≤‖φ‖≤b.
A similar proof immediately yields the next corollary:
Corollary 2.1. Assume that (2.1) is LUAS with radius of attraction δ, that is, there exists a function β∈KL satisfying ‖x(t;φ)‖≤β(‖φ‖,t−t0),∀t≥t0,‖φ‖≤δ. Fix positive scalars a,b,ν such that a≤b≤δ. Then there exists a scalar τ>0 such that β(‖φ‖,t−t0)≤ν‖φ‖ and ‖x(t;φ)‖≤ν‖φ‖, ∀t≥t0+τ,a≤‖φ‖≤b.
This section first investigates the convergence of delayed switched systems with perturbations, and then analyzes stability of switched cascade systems with delays.
Consider the following perturbed system of (2.1)
˙y(t)=fσ(t)(t,yt,d(t))+u(t),t≥t0,y(t)=φ(t),t∈[t0−d,t0], | (3.1) |
with u(t) being perturbation. The next assumption is always imposed on u(t):
Assumption 3.1. ‖u(t)‖≤α1exp(−γ1(t−t0)),∀t≥t0, for some α1>0,γ1>0.
The following lemma estimates the error between the trajectories of (2.1) and (3.1) with the assumption that both systems are with a same initial function.
Lemma 3.1. Assume that systems (2.1) and (3.1) evolve from c≥t0 and are with a same initial function ϕ defined on [c−d,c]. Let x(t;ϕ),y(t;ϕ) be solutions to (2.1) and (3.1), respectively. Define
e(t;c)=y(t;ϕ)−x(t;ϕ),ci=c+i2L,i∈N0,‖u‖i=supci−1≤s≤ci{‖u(s)‖},i∈N. |
The following statements hold:
1. Suppose that Assumption 2.1 holds with ϑ=∞. Then
‖e(t;c)‖≤1Li∑l=12i−l‖u‖l,t∈[ci−1,ci),i∈N. | (3.2) |
2. Suppose that Assumption 2.1 holds with ϑ∈R+. If xs,ys∈Cϑ([−d,0],Rn) hold for any s∈[c,t], then (3.2) is true.
Proof. Item 2 is exactly [14,Lemma 7], and Item 1 naturally holds for ϑ=∞.
Note that the above lemma provides an estimate of difference between solutions to (2.1) and (3.1) on [ci−1,ci), which is completely determined by perturbation u(t) and is not affected by initial condition. Item 1 is a global version and Item 2 is a local one.
Define
μ(t)=2⌈t/L⌉−1Lα1. | (3.3) |
Clearly, μ(t) monotonically increases. It follows from (3.2) that
‖e(t;c)‖≤1L⌈(t−c)/L⌉∑l=12⌈(t−c)/L⌉−lsupc≤s≤t‖u(s)‖=2⌈(t−c)/L⌉−1Lsupc≤s≤t‖u(s)‖, |
which, by Assumption 3.1, further means that
‖e(t;c)‖≤μ(t−c)exp(−γ1(c−t0)),t≥c. | (3.4) |
The identity y(t;φ)=y(t;yc) clearly holds for t≥c≥t0. If (2.1) is GUAS, then there exists a β∈KL satisfying ‖x(t;φ)‖≤β(‖φ‖,t−t0),∀t0≥0,t≥t0, and thus
‖y(t;φ)‖=‖y(t;yc)‖≤‖x(t;yc)‖+‖e(t;c)‖≤β(‖yc‖,t−c)+‖e(t;c)‖,t≥c. |
Hence,
‖y(t;φ)‖≤‖x(t;yc)‖+‖e(t;c)‖,t≥c, | (3.5) |
‖y(t;φ)‖≤β(‖yc‖,0)+‖e(t;c)‖,t≥c, | (3.6) |
(3.5) and (3.6), together with (3.4), indicate that
‖y(t;φ)‖≤‖x(t;yc)‖+μ(t−c)exp(−γ1(c−t0)),t≥c, | (3.7) |
‖y(t;φ)‖≤β(‖yc‖,0)+μ(t−c)exp(−γ1(c−t0)),t≥c. | (3.8) |
Fix ϑ in Assumption 2.1. If (2.1) is LUAS with the radius of attraction δ and if ‖ys‖≤min{δ,ϑ} for s∈[c,t], then (3.5)-(3.8) also hold.
Lemma 3.2. Fix a uniform Si. Suppose that system (2.1) is LUAS. Then the solution to system (3.1) is bounded on [t0,∞) with ‖φ‖≤δ1,α1≤δ1 for some δ1.
iHere we drop the uniform property P from S(P). In the sequel, S(P) will also be denoted by S.
Proof. The importance of uniformity of S is demonstrated first. We are concerned with the dynamics of system (3.1) over given set S and will exploit evolution of system (2.1) with different starting time c>t0 and initial function yc defined on [c−d,c]. Define Sc={σ:[c,∞)→{1,…,m},σ∈S}. Since S is uniform with given uniform P, Sc is necessarily uniform with P. System (2.1) is LUAS implies that there exists a constant ε>0 and a function β∈KL such that ‖x(t;φ)‖≤β(‖φ‖,t−t0),∀t0≥0,t≥t0,‖φ‖≤ε, where φ∈C([t0−d,t0],Rn). It also holds that ‖x(t;ϕ)‖≤β(‖ϕ‖,t−c),∀c>t0,t≥c,‖ϕ‖≤ε with ϕ∈C([c−d,c],Rn) since both S and Sc are uniform with P.
β∈KL implies existence of τ>0 such that
β(ε,t−t0)≤0.5ε,∀t≥t0+τ. | (3.9) |
Let ζ=0.5Lε2⌈(τ+d)/L⌉−1 and take δ1=min{ε,ζ}. Suppose that φ,α1 satisfy ‖φ‖≤δ1,α1≤δ1. By definition of μ(t),
μ(τ+d)≤0.5ε. | (3.10) |
Inequality (3.7) with c=t0 indicates that
‖y(t;φ)‖≤‖x(t;φ)‖+μ(t−t0)≤β(δ1,t−t0)+μ(t−t0),t≥t0, | (3.11) |
(3.9) and the definition of δ1 mean that β(δ1,t−t0)≤β(ε,t−t0), which, together with (3.10), (3.11), and the monotonicity of μ, gives the following estimate:
‖y(t;φ)‖≤β(ε,t−t0)+0.5ε≤ε,t∈[t0+τ,t0+τ+d]. | (3.12) |
By (3.7),
‖y(t;φ)‖≤‖x(t;yt0+τ+d)‖+μ(t−t0−τ−d)exp(−γ1(τ+d)),t≥t0+τ+d. | (3.13) |
The consequence of (3.12) is ‖yt0+τ+d‖≤ε. Clearly, exp(−γ1(τ+d))<1. Therefore, (3.13) implies that
‖y(t;φ)‖≤β(ε,t−t0−τ−d)+μ(τ+d)≤ε,t∈[t0+2τ+d,t0+2τ+2d]. | (3.14) |
Following a similar process, one can show that for any i∈N0, the following inequality holds:
‖y(t;φ)‖≤ε,t∈[t0+(i+1)τ+id,t0+(i+1)τ+(i+1)d]. | (3.15) |
By (3.8) and (3.15), it is not difficult to see that
‖y(t;φ)‖≤β(‖yt0+iτ+id‖,0)+μ(t−t0−iτ−id)exp(−γ1(iτ+id))≤β(ε,0)+μ(τ+d)exp(−γ1(iτ+id))≤β(ε,0)+0.5ε,t∈[t0+iτ+id,t0+(i+1)τ+(i+1)d],i∈N0. | (3.16) |
In a word, if ‖φ‖≤δ1,α1≤δ1, then
‖y(t;φ)‖≤β(ε,0)+0.5ε,t≥t0. | (3.17) |
The proof is completed.
We are in a position to present the following result which shows that the solution to system (3.1) approaches zero asymptotically provided that system (2.1) is LUAS.
Theorem 3.1. Fix a uniform S and consider system (3.1). Suppose that system (2.1) is LUAS and that Assumption 2.1 holds. Then there exists a ˉβ∈KL and a scalar δ1>0 such that
‖y(t;φ)‖≤ˉβ(θ,t−t0),∀t≥t0,t0≥0, | (3.18) |
for any ‖φ‖<δ1,θ<δ1, where θ=max{‖φ‖,α1}.
Proof. Since system (2.1) is LUAS, there exists a β∈KL and ϑ such that Assumption 2.1 is satisfied and that
‖x(t;φ)‖≤β(‖φ‖,t−t0),∀t≥t0,t0≥0,‖φ‖≤ϑ. | (3.19) |
It is easy to see that there exists a unique constant ε>0 such that β(ε,0)+0.5ε=ϑ. According to (3.17) and Lemma 3.2, one can fix δ1>0 such that
‖y(t;φ)‖≤ϑ,∀t≥t0,‖φ‖≤δ1,α1≤δ1, | (3.20) |
which means that δ≜sup‖φ‖≤δ1,α1≤δ1,t≥t0{‖y(t;φ)‖}≤ϑ exists. Fix φ,α1 with ‖φ‖<δ1,α1<δ1 and let θ=max{‖φ‖,α1}. Thus,
‖y(t;φ)‖≤δ,∀t≥t0. | (3.21) |
Since β(δq,0)+0.5δq2=0 if q=0 and β(δq,0)+0.5δq2 is strictly increasing with respect to q, we can pick the unique q∈(0,1) satisfying β(δq,0)+0.5δq2=δ (note that β(δ,0)≥δ).
By Corollary 2.1, there exists a positive increasing sequence {τi}∞i=1 such that
β(s,t−t0)≤0.5qs,∀t0≥0,t≥t0+τi,δqi≤s≤δqi−1, | (3.22) |
which further means
β(s,t−t0)≤0.5δqi,∀t0≥0,t≥t0+τi,s≤δqi−1, | (3.23) |
Denote μi=μ(τi+d) with μ defined in (3.3). Choose a1>0 satisfying μ1θexp(−a1γ1)≤0.5δq. Note that such an a1 does exist, since μ1,θ,γ1,δ and q are all known positive constants. Then, pick the minimal b1∈N and a2>0 such that μ2θexp(−a2γ1)≤0.5δq2 and a2=a1+b1(τ1+d). Construct in the same manner a positive sequence {ai}∞i=1 and a positive integer sequence {bi}∞i=1 which are minimal in the sense that ai(i>1),bi(i≥1) are minimal values satisfying
μiθexp(−aiγ1)≤0.5δqi,ai+1=ai+bi(τi+d),i∈N. | (3.24) |
Note that ai,bi are independent of t0. The following symbols will be used repeatedly.
ci=t0+ai,ail=ai+l(τi+d),cil=t0+ail,i∈N,l∈{0,1,…,bi}Iil=[cil−1,cil],I_il=[cil−1,cil−d],ˉIil=[cil−d,cil],i∈N,l∈{1,…,bi}. | (3.25) |
It is clear that
ai0=ai,aibi=ai+1,ci0=ci,cibi=ci+1,i∈N.
Taking c=cil−1, it follows form (3.4) that
‖e(t;cil−1)‖≤μ(t−cil−1)exp(−γ1(cil−1−t0)),t∈Iil,i∈N,l∈{1,…,bi}. | (3.26) |
Since μ(s) is increasing in s, it holds that μ(t−cil−1)≤μ(cil−cil−1)=μ(τi+d)=μi,∀t∈Iil. This, together with (3.26), implies that
‖e(t;cil−1)‖≤μiexp(−γ1(cil−1−t0))=μiexp(−ail−1γ1),t∈Iil,i∈N,l∈{1,…,bi}. |
By definition of ail in (3.25), ai=ai0<ai1<⋯<aibi. The above inequality means that
‖e(t;cil−1)‖≤μiexp(−aiγ1),t∈Iil,i∈N,l∈{1,…,bi}, |
which, by considering the inequality in (3.24), results in
‖e(t;cil−1)‖≤0.5δqi,t∈Iil,i∈N,l∈{1,…,bi}. | (3.27) |
First prove that
‖y(t;φ)‖≤δq,t∈ˉI1b1. | (3.28) |
Condition (3.21) clearly means that
‖yci‖≤δ,i∈N. | (3.29) |
By the definition of τ1, it holds that β(s,t−t0)≤0.5qs,t≥t0+τ1,δq≤s≤δ. This inequality, combining (3.27) (with i=l=1), (3.29) (with i=1), and (3.5), yields that
‖y(t;φ)‖≤0.5q‖yc1‖+0.5δq≤δq,c11−d≤t≤c11. | (3.30) |
If b1=1 then (3.28) holds; otherwise suppose that ‖y(t;φ)‖≤δq(t∈ˉI1l) with l∈{1,…,b1−1}. (3.7) and (with c=c11) and (3.27) and (with i=1,l=l+1) imply that
‖y(t;φ)‖≤β(‖yc1l‖,t−c1l)+0.5δq≤δq,t∈ˉI1l+1. |
By induction, ‖y(t;φ)‖≤δq,t∈ˉI1l+1 holds for any l∈{1,…,bi}. Therefore (3.28) is true.
Now show that for i∈N∖{1}, it holds that
‖y(t;φ)‖≤β(δqi−1,0)+0.5δqi,ci<t≤ci+1 | (3.31) |
‖y(t;φ)‖≤δqi,t∈ˉIibi. | (3.32) |
Consider (3.31) and (3.32) for the case i=2. By condition (3.28) and the definitions of b1,c2, we have
‖y(t;φ)‖≤β(‖yc2‖,t−c2)+0.5q2δ≤β(qδ,t−c2)+0.5q2δ,t∈I21. | (3.33) |
By the definition of τ2 and considering (3.23) (with i=2), (3.33) further means that
‖y(t;φ)‖≤β(qδ,τ2)+0.5q2δ≤0.5δq2+0.5δq2=δq2,t∈ˉI21. | (3.34) |
If b2=1, then (3.31) and (3.32) hold for i=2. Otherwise, following a reasoning similar to the process from (3.33) to (3.34), and using the mathematical induction principle, it yields that
‖y(t;φ)‖≤β(‖yc2l−1‖,t−c2l−1)+0.5δq2,t∈I2l,l∈{1,…,b2}, | (3.35) |
‖y(t;φ)‖≤q2δ,t∈ˉI2l,l∈{1,…,b2}. | (3.36) |
Since β is decreasing in the second argument, (3.35) and (3.36) respectively imply that (3.31) and (3.32) hold for i=2. Moreover, in a very similar manner proving the case i=2, it is straightforward to verify that (3.31) and (3.32) hold for i+1 provided that they hold for i≥2. Therefore, by the mathematical induction principle, (3.31) and (3.32) hold for any i∈N∖{1}.
Conditions (3.21), (3.31) and the fact β(δq,0)+0.5δq2=δ produce that
‖y(t;φ)‖≤δ,t∈[t0,c2],‖y(t;φ)‖≤β(δqi−1,0)+0.5δqi,t∈(ci,ci+1],i∈N∖{1}. | (3.37) |
Fix a scalar ϵ>0 and introduce
ˉβ(θ,t)={δ+ϵθ,t∈[0,a2],β(δqi−1,0)+0.5(δ+ϵθ)qi,t∈(ai,ai+1],i∈N∖{1}. | (3.38) |
Since δ=sup‖φ‖≤θ,α1≤θ,t≥t0{‖y(t;φ)‖}, δ is increasing in θ. Fix 0≤θ1<θ2,t≥0. If t∈[0,a2], then ˉβ(θ2,t)−ˉβ(θ1,t)≥ϵθ2−ϵθ1>0; if t∈(ai,ai+1] for some i∈N∖{1}, then ˉβ(θ2,t)−ˉβ(θ1,t)>0 also holds. That is, ˉβ(θ,t) strictly monotonically increases in θ. Moreover, ˉβ(θ,t) monotonically decreases in t, and approaches zero as t→∞. Therefore, ˉβ belongs to KL and is independent of t0.
(3.37) and (3.38) indicate that ‖y(t;φ)‖≤ˉβ(θ,t−t0). The proof is completed.
It seems that the constraint ‖u(t)‖≤α1exp(−γ1(t−t0)) in Assumption 3.1 is too restrictive. Actually, in our context, if ‖u(t)‖ is upper bounded by a function asymptotically rather than exponentially decaying to zero, then the perturbed system may diverge, as indicated in the next example.
Example 3.1. Consider the following scalar system:
˙x(t)=a(t)x(t), | (3.39a) |
˙y(t)=a(t)y(t)+u(t). | (3.39b) |
Let a(t)=ln0.5<0,u(t)=1 for t∈[0,1), a(t)=−0.6ln0.5>0 for t∈[1,2),a(t)=ln0.5 for t∈[2,3),u(t)=0.6 for t∈[1,3). Define for i∈N∖{1} the interval Ωi=[2i−1,2i+1−1) and u(t)=0.6i,∀t∈Ωi. Thus, u(t)→0 as t→0. Note that u(t) does not satisfy Assumption 3.1. Further define Ωil=[2i+l−1,2i+l),∀l∈{0,1,…,2i−1}. a(t) is defined on Ωi,i≥2, in the following way:
a(t)={−0.6ln0.5,t∈Ωi0,ln0.5,t∈Ωil,l∈{1,3,…,2i−1},−ln0.5,t∈Ωil,l∈{2,3,…,2i−2}. |
It is not difficult to show that (3.39a) is asymptotically rather than exponentially stable and that (3.39b) diverges. This fact is shown in Figure 1.
Consider the following cascade system
˙x(t)=˜fσ(t)(t,xt,˜d(t)),t≥t0, | (3.40a) |
˙y(t)=fσ(t)(t,yt,d(t))+gσ(t)(t,xt,ˉd(t)),t≥t0, | (3.40b) |
col(x(t),y(t))=φ(t),t∈[t0−d,t0],
where x(t)∈Rn1,y(t)∈Rn2, and φ=col(φ1,φ2), which means that for each t∈[t0−d,t0], φ(t)=col(φ1(t),φ2(t)) with φ1(t)∈Rn1,φ2(t)∈Rn2. ˜d(t),d(t),ˉd(t) are different delay vectors, and d is upper bound of all delays. (3.40a) and
˙y(t)=fσ(t)(t,yt,d(t)),y(t)=φ2(t),t∈[t0−d,t0], | (3.41) |
are two separate systems of system (3.40), and gσ(t)(t,xt,ˉd(t)) in (3.40b) is the coupling term. Note that the state of system (3.40) is col(x(t),y(t)). As usual, it is assumed that ˜fl(⋅,0,⋅)=0,fl(⋅,0,⋅)=0,l∈{1,…,m}.
Assumption 3.2. There exist two positive scalars L,ϑ such that
‖gl(⋅,x,⋅)‖≤L‖x‖,∀x∈Cϑ([−d,0],Rn1). | (3.42) |
The following result is a consequence of Theorem 3.1.
Theorem 3.2. Fix a uniform S. Suppose that Assumption 3.2 holds with ϑ∈R+ and that ˜fl,fl are continuous and are locally Lipschitz in the second argument, uniformly in the first and third ones. System (3.40) is LUAS if (3.40a) is LUES and (3.41) is LUAS.
Proof. Let us view (3.41) and (3.40b) as the nominal system (2.1) and the perturbed system (3.1) with perturbation u(t)=gσ(t)(t,xt,ˉd(t)) in Theorem 3.1, respectively.
Let ϑ,L be as in Assumption 3.2. System (3.40a) being LUES means that there exist δ1>0,α≥1\footnoteii and γ>0 such that ‖x(t;φ1)‖≤αexp(−γ(t−t0))‖φ1‖,∀t≥t0,‖φ1‖≤δ1. If we choose φ1 satisfying ‖φ1‖≤min{ϑαexp(γd),δ1α} then ‖x(t;φ1)‖≤δ1,∀t≥t0. As a result, ‖xt‖≤αexp(−γ(t−t0−d))‖φ1‖≤αexp(γd)‖φ1‖≤ϑ, ∀t≥t0. By Assumption 3.2,
iiBy definition of exponential stability, α>0. By the continuous dependence of solution on initial function, it is easy to that α≥1 holds (for example, take φ as a nonzero constant function).
‖gσ(t)(t,xt,ˉd(t))‖≤Lαexp(−γ(t−t0−d))‖φ1‖=ς‖φ1‖exp(−γ(t−t0)),∀t≥t0, | (3.43) |
where ς=Lαexp(γd).
Note that (3.41) is LUAS. It follows from (3.43) and Theorem 3.1 that there exists a constant δ2 and a β∈KL such that ‖y(t;φ2)‖≤β(max{‖φ2‖,ς‖φ1‖},t−t0) for ‖φ2‖<δ2,ς‖φ1‖<δ2,∀t≥t0, where y(t;φ2) is the solution to (3.40b).
Now take δ=min{δ2,δ2ς}. Fix φ=col(φ1,φ2) with ‖φ‖<δ. It follows that
‖col(x(t;φ),y(t;φ))‖≤‖x(t;φ)‖+‖y(t;φ)‖≤αexp(−γ(t−t0))‖φ1‖+β(max{‖φ2‖,ς‖φ1‖},t−t0)≤αexp(−γ(t−t0))‖φ‖+β(max{‖φ‖,ς‖φ‖},t−t0)≤αexp(−γ(t−t0))‖φ‖+β(max{1,ς}‖φ‖,t−t0), ∀t≥t0. | (3.44) |
Function αexp(−γ(t−t0))‖φ‖+β(max{1,ς}‖φ‖,t−t0) belongs to KL. The proof is completed.
It is worth pointing out that [14,Corollary 18] also holds for system (3.40) with uniform S. This conclusion follows from a proof line similar to Theorem 3.2. Combining this conclusion and Theorem 3.2 above, one has the next corollary:
Corollary 3.1. Fix a uniform S. Suppose that Assumption 3.2 holds and that ˜fl,fl are continuous and are locally Lipschitz in the second argument, uniformly in the first and third ones. System (3.40) is LUAS if one of the following statements holds:
1. (3.40a) is LUES and (3.41) is LUAS.
2. (3.40a) is LUAS and (3.41) is LUES.
Remark 3.1. One special case of switched systems is non-switched systems, that is, there exists only one subsystem. All the main results in the present paper are valid for non-switched systems.
Remark 3.2. The main results presented in Corollary 3.1, can be easily employed for designing controller. Consider the following control system
˙x(t)=˜fσ(t)(t,xt,˜d(t))+˜v(t), | (3.45a) |
˙y(t)=fσ(t)(t,yt,d(t))+gσ(t)(t,xt,ˉd(t))+v(t), | (3.45b) |
with \tilde{\boldsymbol{v}}(t), \boldsymbol{v}(t) being control inputs and \boldsymbol{g}_{\sigma(t)} satisfying Assumption 3.2. To design a controller making (3.45) asymptotically stable, it suffices to design \tilde{\boldsymbol{v}}(t), \boldsymbol{v}(t) such that (3.45a) is uniformly asymptotically (exponentially) stable and \dot{\boldsymbol{y}}(t) = \boldsymbol{f}_{\sigma(t)}\left(t, \boldsymbol{y}_{t}, \boldsymbol{d}(t)\right)+\boldsymbol{v}(t) is uniformly exponentially (asymptotically) stable, without paying any attention to the term \boldsymbol{g}_{\sigma(t)}\left(t, \boldsymbol{x}_{t}, \bar{\boldsymbol{d}}(t)\right) .
Remark 3.3. It is assumed in Theorem 3.2 that system (3.41) is uniformly asymptotically stable. In this case, Theorem 3.2 can only provide a local stability condition, leaving the global one open. If system (3.41) is uniformly exponentially stable, then both local and global stability conditions can be established, for details see [14,Corollary 18].
This section provides an example to demonstrate Theorem 3.2.
Example 4.1. Consider the following system which is a reduced version of (3.40):
\begin{align} \dot{\boldsymbol{x}}(t) = \;&\tilde{\boldsymbol{f}}_{\sigma(t)}\left(\boldsymbol{x}(t), \boldsymbol{x}(t-d)\right), \end{align} | (4.1a) |
\begin{align} \dot{\boldsymbol{y}}(t) = \;&\boldsymbol{f}_{\sigma(t)}\left(\boldsymbol{y}(t), \boldsymbol{y}(t-d)\right)+ \boldsymbol{g}\left(\boldsymbol{x}(t), \boldsymbol{x}(t-d)\right), \end{align} | (4.1b) |
where \sigma: \left[t_0, \infty\right)\rightarrow \left\{1, 2\right\} , \boldsymbol{x}(t) = \mathrm{col}\left(x _1(t), x _2(t)\right)\in\mathbb{R}^2, \boldsymbol{y}(t) = \mathrm{col}\left(y_1(t), y_2(t)\right)\in\mathbb{R}^2, and
\begin{aligned} \tilde{\boldsymbol{f}}_{1}(\boldsymbol{x}, \boldsymbol{y})=&\left[\begin{array}{cc} -5-0.1 \sin x_{1} & -1+0.2 \sin x_{2} \\ 1+0.1 \cos y_{1} & -4-0.3 \cos y_{2} \end{array}\right] \boldsymbol{x} \\ &+\left[\begin{array}{cc} -0.06+0.1 \sin y_{1} & 0.04-0.2 \sin y_{2} \\ 0.02-0.2 \cos x_{1} & -0.02-0.1 & \cos x_{2} \end{array}\right] \boldsymbol{y}, \\ \tilde{\boldsymbol{f}}_{2}(\boldsymbol{x}, \boldsymbol{y})=&\left[\begin{array}{ll} -3+0.4 \cos x_{1} & 2+0.2 \cos x_{2} \\ -1-0.1 \sin y_{1} & -3.8+0.2 \sin y_{2} \end{array}\right] \boldsymbol{x} \\ &+\left[\begin{array}{cc} 0.02+0.1 \cos y_{1} & 0.04-0.2 \sin y_{2} \\ -0.06-0.2 \cos x_{1} & 0.02-0.1 \cos x_{2} \end{array}\right] \boldsymbol{y}, \\ \tilde{\boldsymbol{f}}_{1}(\boldsymbol{x}, \boldsymbol{y})=&\left[\begin{array}{cc} -2 & -1 \\ 1 & -3 \end{array}\right] \boldsymbol{x}+\left[\begin{array}{cc} -0.6 & 0.4 \\ 0.8 & -0.5 \end{array}\right] \boldsymbol{y}, \\ \tilde{\boldsymbol{f}}_{2}(\boldsymbol{x}, \boldsymbol{y})=&\left[\begin{array}{cc} -3 & 2 \\ -2 & -4 \end{array}\right] \boldsymbol{x}+\left[\begin{array}{cc} 0.7 & 0.3 \\ -0.4 & 0.5 \end{array}\right] \boldsymbol{y}, \\ \tilde{\boldsymbol{g}}(\boldsymbol{x}, \boldsymbol{y})=& \operatorname{col}\left(y_{2} \sin x_{2}, y_{1} \cos x_{1}\right) \\ \tilde{\boldsymbol{x}}=& \operatorname{col}\left(x_{1}, x_{2}\right), \boldsymbol{y}=\operatorname{col}\left(y_{1}, y_{2}\right) \in \mathbb{R}^{2}. \end{aligned} |
By [31,Theorem 1], system (4.1a) is exponentially stable with d = 2 under arbitrary switching signals. Moreover, it follows from [32] that system \dot{\boldsymbol{y}}(t) = \boldsymbol{f}_{\sigma(t)}\left(\boldsymbol{y}(t), \boldsymbol{y}(t-d)\right) is asymptotically stable with d = 2 under arbitrary switching signals.
By Theorem 3.2, system (4.1) is asymptotically stable under arbitrary switching signals; this fact is shown in Figures 2 and the involved switching signal is plotted in Figures 3.
This paper has addressed the asymptotic stability issue of continuous-time switched cascade nonlinear systems with delays. Technically, the main results rely on the robust convergence property of delayed switched nonlinear systems with perturbations. It was shown that trajectory of the perturbed system asymptotically approaches origin if the perturbation can be upper bounded by an exponentially decaying function and if the nominal system is asymptotically stable. This property was then employed to analyse the asymptotic stability of switched cascade nonlinear systems with delays, and some stability conditions have been proposed. Two points should be pointed out: (ⅰ) The considered delays are bounded. (ⅱ) Perturbations in this paper are additive. Therefore, more challenging work in the future is to investigate dynamics of systems involving unbounded delays and multiplicative perturbations.
This work was partially supported by National Nature Science Foundation (62073270), State Ethnic Affairs Commission Innovation Research Team, Innovative Research Team of the Education Department of Sichuan Province (15TD0050), and Fundamental Research Funds for the Central Universities (2021HQZZ02).
The authors declare there is no conflict of interests.
[1] |
D. Baleanu, A. Jajarmi, H. Mohammadi, S. Rezapour, A new study on the mathematical modelling of human liver with Caputo-Fabrizio fractional derivative, Chaos Soliton. Fract., 134 (2021), 109705. https://doi.org/10.1016/j.chaos.2020.109705 doi: 10.1016/j.chaos.2020.109705
![]() |
[2] |
H. Mohammadi, S. Kumar, S. Rezapour, S. Etemad, A theoretical study of the Caputo-Fabrizio fractional modeling for hearing loss due to Mumps virus with optimal control, Chaos Soliton. Fract., 141 (2021), 110668. https://doi.org/10.1016/j.chaos.2021.110668 doi: 10.1016/j.chaos.2021.110668
![]() |
[3] | A. Din, Y. Li, T. Khan, G. Zaman, Mathematical analysis of spread and control of the novel corona virus (COVID-19) in China, Chaos Soliton. Fract., 141 (2020), 110286. https://doi.org/0.1016/j.chaos.2020.110286 |
[4] |
A. Din, Y. Li, Controlling heroin addiction via age-structured modeling, Adv. Differ. Equations, 2021, 1–17. https://doi.org/10.1186/s13662-020-02983-5 doi: 10.1186/s13662-020-02983-5
![]() |
[5] |
A. Din, Y. Li, A. Yusuf, Delayed hepatitis B epidemic model with stochastic analysis, Chaos Soliton. Fract., 146 (2021), 110839. https://doi.org/10.1016/j.chaos.2021.110839 doi: 10.1016/j.chaos.2021.110839
![]() |
[6] |
S. T. M. Thabet, S. Etemad, S. Rezapour, On a coupled Caputo conformable system of pantograph problems, Turk. J. Math., 45 (2021), 496–519. https://doi.org/10.3906/mat-2010-70 doi: 10.3906/mat-2010-70
![]() |
[7] |
J. J. Nieto, J. Pimentel, Positive solutions of a fractional thermostat model, Bound. Value Probl., 2013 (2013), 5. https://doi.org/10.1186/1687-2770-2013-5 doi: 10.1186/1687-2770-2013-5
![]() |
[8] |
G. Infante, J. Webb, Loss of positivity in a nonlinear scalar heat equation, Nonlinear Differ. Equ. Appl., 13 (2006), 249–261. https://doi.org/10.1007/s00030-005-0039-y doi: 10.1007/s00030-005-0039-y
![]() |
[9] |
D. Baleanu, S. Etemad, S. Rezapour, A hybrid Caputo fractional modeling for thermostat with hybrid boundary value conditions, Bound. Value Probl., 2020 (2020), 64. https://doi.org/10.1186/s13661-020-01361-0 doi: 10.1186/s13661-020-01361-0
![]() |
[10] |
S. Rezapour, M. E. Samei, On the existence of solutions for a multi-singular pointwise defined fractional q-integro-differential equation, Bound. Value Probl., 2020 (2020), 38. https://doi.org/10.1186/s13661-020-01342-3 doi: 10.1186/s13661-020-01342-3
![]() |
[11] |
J. Wang, X. Li, Ulam-Hyers stability of fractional Langevin equations, Appl. Math. Comput., 258 (2015), 72–83. https://doi.org/10.1016/j.amc.2015.01.111 doi: 10.1016/j.amc.2015.01.111
![]() |
[12] |
J. V. D. C. Sousa, E. C. De Oliveira, Ulam-Hyers stability of a nonlinear fractional Volterra integro-differential equation, Appl. Math. Lett., 81 (2018), 50–56. https://doi.org/10.1016/j.aml.2018.01.016 doi: 10.1016/j.aml.2018.01.016
![]() |
[13] |
I. Ahmad, P. Kumam, K. Shah, P. Borisut, K. Sitthithakerngkiet, M. A. Demba, Stability results for implicit fractional pantograph differential equations via \phi-Hilfer fractional derivative with a nonlocal Riemann-Liouville fractional integral condition, Mathematics, 8 (2020), 94. https://doi.org/10.3390/math8010094 doi: 10.3390/math8010094
![]() |
[14] |
B. Ghanbari, Abundant exact solutions to a generalized nonlinear Schrödinger equation with local fractional derivative, Math. Methods Appl. Sci., 44 (2021), 8759–8774. https://doi.org/10.1002/mma.7302 doi: 10.1002/mma.7302
![]() |
[15] |
Q. M. A. Al-Mdallal, An efficient method for solving fractional Sturm-Liouville problems, Chaos Soliton. Fract., 40 (2009), 183–189. https://doi.org/10.1016/j.chaos.2007.07.041 doi: 10.1016/j.chaos.2007.07.041
![]() |
[16] |
B. Ghanbari, On novel nondifferentiable exact solutions to local fractional Gardner's equation using an effective technique, Math. Methods Appl. Sci., 44 (2021), 4673–4685. https://doi.org/10.1002/mma.7060 doi: 10.1002/mma.7060
![]() |
[17] |
E. Bairamov, I. Erdal, S. Yardimci, Spectral properties of an impulsive Sturm-Liouville operator, J. Inequal. Appl., 2018 (2018), 191. https://doi.org/10.1186/s13660-018-1781-0 doi: 10.1186/s13660-018-1781-0
![]() |
[18] |
B. Ghanbari, A new model for investigating the transmission of infectious diseases in a prey-predator system using a non-singular fractional derivative, Math. Methods Appl. Sci., 2021. https://doi.org/10.1002/mma.7412 doi: 10.1002/mma.7412
![]() |
[19] |
D. Baleanu, H. Mohammadi, S. Rezapour, On a nonlinear fractional differential equation on partially ordered metric spaces, Adv. Differ. Equ., 2013 (2013), 83. https://doi.org/10.1186/1687-1847-2013-83 doi: 10.1186/1687-1847-2013-83
![]() |
[20] |
S. Djilali, B. Ghanbari, The influence of an infectious disease on a prey-predator model equipped with a fractional-order derivative, Adv. Differ. Equ., 2021 (2021), 20. https://doi.org/10.1186/s13662-020-03177-9 doi: 10.1186/s13662-020-03177-9
![]() |
[21] |
V. S. Erturk, Computing eigenelements of Sturm-Liouville problems of fractional order via fractional differential transform method, Math. Comput. Appl., 16 (2011), 712–720. https://doi.org/10.3390/mca16030712 doi: 10.3390/mca16030712
![]() |
[22] |
B. Ghanbari, On approximate solutions for a fractional prey–predator model involving the Atangana–Baleanu derivative, Adv. Differ. Equ., 2020 (2020), 679. https://doi.org/10.1186/s13662-020-03140-8 doi: 10.1186/s13662-020-03140-8
![]() |
[23] | A. Lachouri, A. Ardjouni, A. Djoudi, Initial value problems for nonlinear Caputo fractional relaxation differential equations, Khayyam J. Math., 8 (2011), 85–93. |
[24] |
B. Ghanbari, On the modeling of the interaction between tumor growth and the immune system using some new fractional and fractional-fractal operators, Adv. Differ. Equ., 2020 (2020), 585. https://doi.org/10.1186/s13662-020-03040-x doi: 10.1186/s13662-020-03040-x
![]() |
[25] |
Gulalai, A. Ullah, S. Ahmad, M. Inc, Fractal fractional analysis of modified KdV equation under three different kernels, J. Ocean Eng. Sci., 2022. https://doi.org/10.1016/j.joes.2022.04.025 doi: 10.1016/j.joes.2022.04.025
![]() |
[26] |
A. Nabti, B. Ghanbari, Global stability analysis of a fractional SVEIR epidemic model, Math. Methods Appl. Sci., 44 (2021), 8577–8597. https://doi.org/10.1002/mma.7285 doi: 10.1002/mma.7285
![]() |
[27] |
D. Baleanu, S. Rezapour, Z. Saberpour, On fractional integro-differential inclusions via the extended fractional Caputo-Fabrizio derivation, Bound. Value Probl., 2019 (2019), 79. https://doi.org/10.1186/s13661-019-1194-0 doi: 10.1186/s13661-019-1194-0
![]() |
[28] |
B. Ghanbari, A fractional system of delay differential equation with nonsingular kernels in modeling hand-foot-mouth disease, Adv. Differ. Equ., 2020 (2020), 536. https://doi.org/10.1186/s13662-020-02993-3 doi: 10.1186/s13662-020-02993-3
![]() |
[29] |
M. M. Matar, M. I. Abbas, J. Alzabut, M. K. A. Kaabar, S. Etemad, S. Rezapour, Investigation of the p-Laplacian nonperiodic nonlinear boundary value problem via generalized Caputo fractional derivatives, Adv. Differ. Equ., 2021 (2021), 68. https://doi.org/10.1186/s13662-021-03228-9 doi: 10.1186/s13662-021-03228-9
![]() |
[30] |
A. M. Yang, Y. Han, J. Li, W. X. Liu, On steady heat flow problem involving Yang-Srivastava-Machado fractional derivative without singular kernel, Therm. Sci., 20 (2017), 717–721. https://doi.org/10.2298/TSCI16S3717Y doi: 10.2298/TSCI16S3717Y
![]() |
[31] | M. A. Al-Gwaiz, Sturm-Liouville theory and its applications, Springer, 2008. https://doi.org/10.1007/978-1-84628-972-9 |
[32] | P. F. Gora, The theory of Brownian motion: A hundred years' anniversary, The 19th Marian Smoluchowski Symposium on Statistical Physics, 2006, 52–57. |
[33] | P. Langevin, On the theory of Brownian motion, Compt. Rendus, 146 (1908), 530–533. |
[34] | R. M. Mazo, Brownian motion: Fluctuations, dynamics, and applications, Oxford University Press, 2002. |
[35] | N. Wax, Selected papers on noise and stochastic processes, Dover, New York, 1954. |
[36] | R. Zwanzig, Nonequilibrium statistical mechanics, Oxford University Press, 2001. |
[37] |
V. Kobelev, E. Romanov, Fractional Langevin equation to describe anomalous diffusion, Prog. Theor. Phys. Supp., 139 (2000), 470–476. https://doi.org/10.1143/PTPS.139.470 doi: 10.1143/PTPS.139.470
![]() |
[38] |
B. J. west, M. Latka, Fractional Langevin model of gait variability, J. NeuroEng. Rehabil., 2 (2005), 24. https://doi.org/10.1186/1743-0003-2-24 doi: 10.1186/1743-0003-2-24
![]() |
[39] |
S. Picozzi, B. J. West, Fractional Langevin model of memory in financial markets, Phys. Rev. E, 66 (2002), 046118. https://doi.org/10.1103/PhysRevE.66.046118 doi: 10.1103/PhysRevE.66.046118
![]() |
[40] |
A. H. Bhrawy, M. A. Alghamdi, A shifted Jacobi-Gauss-Lobatto collocation method for solving nonlinear fractional Langevin equation involving two fractional orders in different intervals, Bound. Value Probl., 2012 (2012), 62. https://doi.org/10.1186/1687-2770-2012-62 doi: 10.1186/1687-2770-2012-62
![]() |
[41] |
B. Ahmad, J. J. Nieto, A. Alsaedi, M. El-Shahed, A study of nonlinear Langevin equation involving two fractional orders in different intervals, Nonlinear Anal.: Real World Appl., 13 (2012), 599–606. https://doi.org/10.1016/j.nonrwa.2011.07.052 doi: 10.1016/j.nonrwa.2011.07.052
![]() |
[42] |
J. Wang, S. Peng, D. O'Rregan, Local stable manifold of Langevin differential equations with two fractional derivatives, Adv. Differ. Equations, 2017 (2017), 335. https://doi.org/10.1186/s13662-017-1389-6 doi: 10.1186/s13662-017-1389-6
![]() |
[43] |
C. Zhai, P. Li, H. Li, Single upper-solution or lower-solution method for Langevin equations with two fractional orders, Adv. Differ. Equations, 2018 (2018), 360. https://doi.org/10.1186/s13662-018-1837-y doi: 10.1186/s13662-018-1837-y
![]() |
[44] |
A. Zada, R. Rizwan, J. Xu, Z. Fu, On implicit impulsive Langevin equation involving mixed order derivatives, Adv. Differ. Equations, 2019 (2019), 489. https://doi.org/10.1186/s13662-019-2408-6 doi: 10.1186/s13662-019-2408-6
![]() |
[45] |
S. Yang, M. Deng, R. Ren, Stochastic resonance of fractional-order Langevin equation driven by periodic modulated noise with mass fluctuation, Adv. Differ. Equations, 2020 (2020), 81. https://doi.org/10.1186/s13662-020-2492-7 doi: 10.1186/s13662-020-2492-7
![]() |
[46] |
W. Sudsutad, K. S. Ntouyas, J. Tariboon, Systems of fractional Langevin equations of Riemann-Liouville and Hadamard types, Adv. Differ. Equations, 2015 (2015), 235. https://doi.org/10.1186/s13662-015-0566-8 doi: 10.1186/s13662-015-0566-8
![]() |
[47] |
J. Tariboon, S. K. Ntouyas, Nonlinear second-order impulsive q-difference Langevin equation with boundary conditions, Bound. Value Probl., 2014 (2014), 85. https://doi.org/10.1186/1687-2770-2014-85 doi: 10.1186/1687-2770-2014-85
![]() |
[48] |
S. I. Denisov, H. Kantz, P. Hanggi, Langevin equation with super-heavy-tailed nois, J. Phys. A: Math. Theor., 43, (2010), 285004. https://doi.org/10.1088/1751-8113/43/28/285004 doi: 10.1088/1751-8113/43/28/285004
![]() |
[49] |
S. C. Lim, M. Li, L. P. Teo, Langevin equation with two fractional orders, Phys. Lett. A, 372 (2008), 6309–6320. https://doi.org/10.1016/j.physleta.2008.08.045 doi: 10.1016/j.physleta.2008.08.045
![]() |
[50] |
M. Uranagase, T. Munakata, Generalized Langevin equation revisited: Mechanical random force and self-consistent structure, J. Phys. A: Math. Theor., 43 (2010), 455003. https://doi.org/10.1088/1751-8113/43/45/455003 doi: 10.1088/1751-8113/43/45/455003
![]() |
[51] |
Z. Heydarpour, J. Izadi, R. George, M. Ghaderi, S. Rezapour, On a partial fractional hybrid version of generalized Sturm-Liouville-Langevin equation, Fractal Fract., 6 (2022), 269. https://doi.org/10.3390/fractalfract6050269 doi: 10.3390/fractalfract6050269
![]() |
[52] |
H. Fazli, H. G. Sun, J. J. Nieto, Fractional Langevin equation involving two fractional orders: Existence and uniqueness revisited, Mathematics, 8 (2020), 743. https://doi.org/10.3390/math8050743 doi: 10.3390/math8050743
![]() |
[53] | B. C. Dhage, On a-condensing mappings in Banach algebras, Math. Stud.-India, 63 (1994), 146–152. |
[54] | B. C. Dhage, A nonlinear alternative in Banach algebras with applications to functional differential equations, Nonlinear Funct. Anal. Appl, 8 (2004), 563–575. |
[55] | B. C. Dhage, Fixed point theorems in ordered Banach algebras and applications, Panam. Math. J., 9 (1999), 83–102. |
[56] |
B. C. Dhage, V. Lakshmikantham, Basic results on hybrid differential equations, Nonlinear Anal.: Hybrid Syst., 4 (2010), 414–424. https://doi.org/10.1016/j.nahs.2009.10.005 doi: 10.1016/j.nahs.2009.10.005
![]() |
[57] |
B. C. Dhage, V. Lakshmikantham, Quadratic perturbations of periodic boundary value problems of second order ordinary differential equations, Differ. Equ. Appl., 2 (2010), 465–486. https://dx.doi.org/10.7153/dea-02-28 doi: 10.7153/dea-02-28
![]() |
[58] |
M. A. E. Herzallah, D. Baleanu, On fractional order hybrid differential equations, Abstr. Appl. Anal., 2014 (2014), 389386. https://doi.org/10.1155/2014/389386 doi: 10.1155/2014/389386
![]() |
[59] |
Y. Zhao, S. Sun, Z. Han, Q. Li, Theory of fractional hybrid differential equations, Comput. Math. Appl., 62 (2011), 1312–1324. https://doi.org/10.1016/j.camwa.2011.03.041 doi: 10.1016/j.camwa.2011.03.041
![]() |
[60] |
H. Ge, J. Xin, On the existence of a mild solution for impulsive hybrid fractional differential equations, Adv. Differ. Equations, 2014 (2014), 211. https://doi.org/10.1186/1687-1847-2014-211 doi: 10.1186/1687-1847-2014-211
![]() |
[61] |
C. Derbazi, H. Hammouche, M. Benchohra, Y. Zhou, Fractional hybrid differential equations with three-point boundary hybrid conditions, Adv. Differ. Equations, 2019 (2019), 125. https://doi.org/10.1186/s13662-019-2067-7 doi: 10.1186/s13662-019-2067-7
![]() |
[62] | A. A. Kilbas, H. M. Srivastava, J. J. Trujillo, Theory and applications of fractional differential equations, Vol. 204, Elsevier, 2006. |
[63] | I. Podlubny, Fractional differential equations, San Diego: Academic Press, 1999. |
[64] | S. G. Samko, A. A. Kilbas, O. I. Marichev, Fractional integral and derivative: Theory and applications, Switzerland: Gordon and Breach Science Publishers, 1993. |
[65] | T. R. Prabhakar, A singular integral equation with a generalized Mittag-Leffler function in the kernel, Yokohama Mathematical Journal, Vol. 19, 1971. |
[66] |
B. Samet, C. Vetro, P. Vetro, Fixed point theorem for \alpha-\psi-contractive type mappings, Nonlinear Anal.: Theory Methods Appl., 75 (2012), 2154–2165. https://doi.org/10.1016/j.na.2011.10.014 doi: 10.1016/j.na.2011.10.014
![]() |
1. | Bangxin Jiang, Yijun Lou, Jianquan Lu, Input-to-state stability of delayed systems with bounded-delay impulses, 2022, 2, 2767-8946, 44, 10.3934/mmc.2022006 | |
2. | Zhifeng Lu, Fei Wang, Yujuan Tian, Yaping Li, Lag synchronization of complex-valued interval neural networks via distributed delayed impulsive control, 2022, 8, 2473-6988, 5502, 10.3934/math.2023277 | |
3. | Ramalingam Sakthivel, Palanisamy Selvaraj, Yeong-Jae Kim, Dong-Hoon Lee, Oh-Min Kwon, Rathinasamy Sakthivel, Robust H_\infty resilient event-triggered control design for T-S fuzzy systems, 2022, 15, 1937-1632, 3297, 10.3934/dcdss.2022028 | |
4. | Zhaoxia Duan, Jinling Liang, Zhengrong Xiang, Filter design for continuous-discrete Takagi-Sugeno fuzzy system with finite frequency specifications, 2023, 3, 2767-8946, 387, 10.3934/mmc.2023031 |