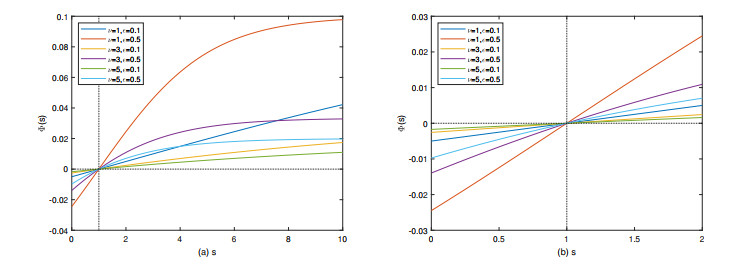
Citation: Wei Zhang, Sheng Cao, Jessica L. Martin, Joachim D. Mueller, Louis M. Mansky. Morphology and ultrastructure of retrovirus particles[J]. AIMS Biophysics, 2015, 2(3): 343-369. doi: 10.3934/biophy.2015.3.343
[1] | Zhizhou Ma, Yujun Qi, Weiqing Liu . A comparative study of pull-out performance of bolted joints in pultruded FRP with drilled holes or punched holes. Mathematical Biosciences and Engineering, 2019, 16(5): 4213-4228. doi: 10.3934/mbe.2019210 |
[2] | Peng Jing, Weichao Wang, Chengxi Jiang, Ye Zha, Baixu Ming . Determinants of switching behavior to wear helmets when riding e-bikes, a two-step SEM-ANFIS approach. Mathematical Biosciences and Engineering, 2023, 20(5): 9135-9158. doi: 10.3934/mbe.2023401 |
[3] | Linxuan Zhou, Jingwei Gao, Qiao Li, Cheng Hu . Simulation study on tractive performance of off-road tire based on discrete element method. Mathematical Biosciences and Engineering, 2020, 17(4): 3869-3893. doi: 10.3934/mbe.2020215 |
[4] | Yuriy Stoyan, Georgiy Yaskov, Tatiana Romanova, Igor Litvinchev, Sergey Yakovlev, José Manuel Velarde Cantú . Optimized packing multidimensional hyperspheres: a unified approach. Mathematical Biosciences and Engineering, 2020, 17(6): 6601-6630. doi: 10.3934/mbe.2020344 |
[5] | Guirong Jiang, Qishao Lu, Linping Peng . Impulsive Ecological Control Of A Stage-Structured Pest Management System. Mathematical Biosciences and Engineering, 2005, 2(2): 329-344. doi: 10.3934/mbe.2005.2.329 |
[6] | Jiaxin Nan, Wanbiao Ma . Stability and persistence analysis of a microorganism flocculation model with infinite delay. Mathematical Biosciences and Engineering, 2023, 20(6): 10815-10827. doi: 10.3934/mbe.2023480 |
[7] | Dongmei Zhang . Unveiling dynamics of urbanization, rural logistics, and carbon emissions: A study based on China's empirical data. Mathematical Biosciences and Engineering, 2024, 21(2): 2731-2752. doi: 10.3934/mbe.2024121 |
[8] | Hongying Shu, Wanxiao Xu, Zenghui Hao . Global dynamics of an immunosuppressive infection model with stage structure. Mathematical Biosciences and Engineering, 2020, 17(3): 2082-2102. doi: 10.3934/mbe.2020111 |
[9] | Hui Li, Mengyao Zhang, Chenbo Zeng . Circular Jaccard distance based multi-solution optimization for traveling salesman problems. Mathematical Biosciences and Engineering, 2022, 19(5): 4458-4480. doi: 10.3934/mbe.2022206 |
[10] | Guangxun Sun, Binxiang Dai . Stability and bifurcation of a delayed diffusive predator-prey system with food-limited and nonlinear harvesting. Mathematical Biosciences and Engineering, 2020, 17(4): 3520-3552. doi: 10.3934/mbe.2020199 |
The spread of infectious diseases threatens people's health and social stability. To control the spread of the epidemic, governments usually take some measures, such as developing vaccines and isolating patients carrying the virus. Before the successful promotion of the vaccine, the government's non-pharmaceutical control measures, which reduce people's contact rate through lockdown and isolation, play a leading role in controlling the epidemic. However, it cannot relieve people's fear of the virus and may deteriorate the national or regional economy [1,2].
Mathematical models are useful tools to analyze the formation of social and economic phenomena under various backgrounds. In recent years, the Boltzmann-type equation, which uses the collision theory to study the statistical distribution of rarefied gas molecules [3], is adopted to investigate the socio-economic phenomena of multi-agent systems, such as opinion formation and wealth distribution (see [4,5,6,7,8]). To discover the socio-economic impact of epidemics, researchers extend the classical Susceptible-Infectious-Recovered (SIR) epidemic model [9,10,11,12], in which agents are separated into three categories: susceptible (S), infected (I) and recovered (R), and integrate the SIR model with the socio-economic phenomena of multi-agent systems, such as people's social contact and wealth exchange behavior [13,14].
The description of socio-economic features involves knowledge of mathematics, sociology, economics, psychology and other fields [15,16,17,18]. In the physical-economic dynamic models, the wealth distribution is determined by the continuous trading features of a large number of agents according to a wealth exchange mechanism, which is characterized by the saving (exchange) propensity of agents and uncertain elements. The uncertainty includes market risks and speculative risks [5]. Saving means that agents are risk-conscious and only use part of their wealth for trading. The saving (exchange) propensity reflects agents' trading decision-making. Decision-making problems are common in life, such as commodity selection, product evaluation, company management, etc. (see [19,20,21]). Persons' decision-making behaviors are affected by psychological preferences, health status, wealth and other factors (see [5,13,22,23,24,25,26]).
Dimarco et al. [13] introduce a kinetic system consisting of three Boltzmann-type equations to describe how a wealth distribution might evolve under the course of an epidemic. In the kinetic system, the transport parts reflect the movement of individuals in different infection statuses and the Boltzmann collision operators depict the change in wealth distributions caused by the wealth exchange behaviors. The wealth exchange mechanism is depicted by the Cordier-Pareschi-Toscani (CPT) model
$ w′=(1−PL)w+PJw∗+ηLJw, $
|
(1.1a) |
$ w′∗=(1−PJ)w∗+PLw+˜ηLJw∗. $
|
(1.1b) |
In (1.1), the pre-trade wealth $ (w, w_{*}) $ of two agents with infection status $ L, J\in\{S, I, R\} $ becomes $ (w^{'}, w_{*}^{'}) $ after the transaction, and the constant $ P_{J}\in[0, 1] $ is exchange propensity for the class of agents with the infectious state $ J $. The random variables $ \eta_{LJ} $ and $ \tilde{\eta}_{LJ} $ stand for the uncertain impact of market risks. Dimarco et al. [13] find that epidemics affect wealth distribution.
In [13], agents in the same infection state have the same exchange propensity (see (1.1)) without considering the psychological differences of people when trading. In addition, the impact of government policies and measures on wealth distribution is not considered. Based on the work in [13], the motivation in our work includes two points. Firstly, we explore wealth distribution when the agent's trading decision is influenced by psychology. Secondly, we discuss the impact of contact control measures and vaccination on wealth distribution.
In a speculative market with one stock and two populations of chartists and fundamentalists, Maldarella and Pareschi [27] consider the influence of agents' behavioral and psychological factors on their investment. The psychological impact is represented by an appropriate value function corresponding to the prospect theory in Kahneman and Tversky [28]. The prospect theory is applied to dynamic models of social and biological phenomena, such as social contacts, alcohol consumption, the age of first marriage, the size of cities, tumor growth, etc. (see [14,29,30,31]). In these studies, the micro modification of the social trait $ x $ is expressed as a single interaction model
$ x∗=x−Φϵδ(xˉx)x+ηx, $
|
(1.2) |
where $ x_{*} $ denotes the value of the trait after the interaction, and $ \eta $ is a random variable with mean zero and bounded variance. For the variable $ s = x/\bar{x} $, the general function $ \Phi_{\delta}^{\epsilon}(s) $ is given by
$ Φϵδ(s)=μeϵ(sδ−1)/δ−1eϵ(sδ−1)/δ+1. $
|
(1.3) |
The constant parameters $ \bar{x} $, $ \mu\in(0, 1) $, $ \delta\in[-1, 1] $ and $ \epsilon > 0 $ are related to the phenomenon in society.
The parameter $ \delta $ plays a key role in determining the properties of the value function (1.3) and the trait's macroscopic features under the updating rule (1.2). Provided that $ \delta\mapsto 0 $, the value function (1.3) is concave, and the stationary solution of the Boltzmann-type kinetic model is a lognormal density describing situations of call center service time and human behaviors (see [30,32,33]). When $ \delta\neq 0 $, (1.3) possesses properties of the prospect theory in [28,34]. For example, the value function (1.3) is concave above $ \bar{x} $ and convex below it. In the case of $ \delta > 0 $, the stationary distribution of the trait $ x $ is a generalized gamma distribution depicting the alcohol consumption in [29]. $ \delta < 0 $ leads to an Amoroso-type distribution, which depicts the formation of social elites caused by social climbing activities [35].
In this paper, to study the influence of epidemics and the psychology of agents on wealth distribution, we will generalize the CPT model (1.1) in [13]. Assuming that agents' trading behavior is affected by their characteristics (such as health state and wealth level) and the prevalence of infectious diseases, we extend the constant exchange propensity of (1.1) as a function of the infected fraction and wealth. Firstly, as a response to the severity of the epidemic, we multiply the constant exchange propensity $ P_{J} $ by a function of the infected fraction at time $ t $ to describe a possible way for agents to reduce the exchange propensity $ P_{J} $. Secondly, considering that the rich have better anti-risk abilities than the poor, unlike the assumption that agents in the same infection state have the same trading propensity in [13], in this paper, we assume that the rich tend to use more wealth for trading to obtain better life enjoyment or investment return, while the poor tend to save wealth to ensure future life needs. We adopt a function $ \Phi(\cdot) $ (see (2.4)), which is a generalized form of (1.3) with $ \delta = 1 $, as a component of the exchange propensity to represent the disturbance of these psychological factors when making trading decisions. We introduce an additional parameter on the denominator of (1.3) to avoid the extreme situation that the increased investment proportion is too large (small) when the wealth level is high (low).
The stationary solution of the conservative socio-economic dynamic model, whose form is determined by the micro-interaction rules, plays a significant role in describing the large-time behavior of the trait under investigation. In this work, we utilize the Boltzmann system [13] to describe the evolutions of wealth distributions, and generalize the constant contact rate in [13] to a time-dependent function. Under the modified binary wealth exchange mechanism proposed in this paper, the Boltzmann system is transformed into the Fokker-Planck equations with quadratic drift and diffusion coefficients by using the quasi-invariant asymptotic limit method [7]. Specifically, the stationary wealth distribution of susceptible (recovered) agents $ f_{L}^{\infty}(w) $, $ L\in \{S, R\} $ in this work satisfies an ordinary differential equation in the form
$ σ2d2dw2[w2f∞L(w)]+ddw[(bw2+˜aLw−c)f∞L(w)]=0, $
|
(1.4) |
where the non-negative coefficients $ \sigma $, $ \tilde{a}_{L} $, $ b $, and $ c > 0 $ rely on the parameters involved in the exchange rule and the epidemic environment. The solution of (1.4) is in the form of the product of an inverse gamma function and an exponential function. Since the microscopic interaction of the trait (alcohol consumption) in [29] is not a binary interaction, the stationary distribution in [29] satisfies Eq (1.4) with $ b\neq0 $ and $ c = 0 $. The stationary distribution in [13] satisfies Eq (1.4) with $ b = 0 $ and $ c\neq0 $. Compared with the work in [13], in our work, the new quadratic term $ b w^{2} $ in the drift coefficient of (1.4) is due to the nonlinear value function $ \Phi(\cdot) $ adopted in the exchange propensity.
The contributions of this paper are summarized as follows. 1) We supplement the exchange propensity form in the wealth distribution literature. Compared with the constant exchange propensity (see [6,13,22,36,37]), the varying exchange propensity in our model reflects people's psychological features. Compared with the exchange propensity in [5], which is a monotone function of wealth, we take into account the asymmetry of the agent's trading psychology at different wealth levels. 2) We supplement the description of the steady-state wealth distribution function in the literature. Polk and Boghosian [38] point out that the expression form of the wealth distribution's tail is not universal. Based on the classical wealth exchange CPT model, if the dynamic model is involved in the feedback control, taxation and redistribution implemented by the government, or the heterogeneity of agents, the steady-state wealth distributions are inverse gamma function (see [5,6,13,22,39,40]). Compared with the inverse gamma wealth distribution obtained in [13], the presence of the exponential function part in the solution of (1.4) makes the wealth distribution curve decay faster as $ w\mapsto\infty $, namely, the slimmer of the tail. This phenomenon corresponds to the decline in the number of super-rich. 3) We verify that the epidemic affects wealth distribution, contact control measures can curb the epidemic and aggravate wealth inequality. Ashraf [41] finds that the increase of COVID-19 confirmed cases has a negative impact on the stock market. Ashraf [2] discovers that social distancing measures are bad for the economy. Dosi et al. [42] point out that COVID-19 and contact controls may aggravate wealth inequality. 4) We illustrate that vaccination can curb the spread of epidemics and help to improve the economy. Hansen and Mano [43] find that vaccination can increase the mobility of the workplace, help to improve the economy and reduce inequality.
The rest of the paper is constructed as follows. In Section 2, we set up the Boltzmann-type system describing the evolution of wealth distribution involving an epidemic environment, and introduce a wealth exchange mechanism containing the environment and agents' psychology. The macroscopic properties of the wealth exchange model, such as the proportion of each group population and their mean wealth, are discussed in Section 3. We obtain a Fokker-Planck system to describe the evolution of wealth distributions in Section 4, and acquire the steady-state wealth distribution under the background of the SIR model. In Section 5, we introduce the government's contact control measures and vaccination into the Boltzmann-type system of wealth distributions and discuss their impact on epidemics and wealth distributions. In addition, we numerically analyze the effect of agents' trading psychology on wealth distribution and verify the existence of bimodal stationary wealth distribution. Conclusions are given in Section 6.
In this paper, under the framework of the SIR epidemic model [10,11], we investigate how wealth distribution might evolve under the course of an epidemic. In the SIR model [11], agents are separated into the susceptible (S), infected (I), and recovered (R) categories. The susceptible group refers to individuals who have no immunity and may be infected by the disease, the infected group includes those who are infectious, and the recovered group includes those who are cured or permanently immunized.
As assumed in the wealth exchange dynamics in [5], we regard that agents are indistinguishable. In addition to the difference of infection status, each agent's state at any time $ t $ is characterized by wealth $ w\geq 0 $. We denote $ \Omega = \{S, I, R\} $. Let $ f_{L}(w, t) $ with $ L\in \Omega $ represent the wealth distribution of agents in group $ L $ at time $ t $. In the SIR epidemic model, the birth and mortality factors are not considered, which is a simplification of the actual epidemic dynamics. Then, the whole agent system's wealth distribution is $ f(w, t) = \sum\limits_{L\in \Omega}f_{L}(w, t) $, and satisfies the normalization condition $ \int_{\mathbb{R}_{+}} f(w, t)dw = 1 $.
Following the idea of wealth exchange dynamics in [13], the wealth distribution $ f_{L}(w, t) $, $ L\in \Omega $ satisfies an integro-differential system of three Boltzmann-type equations
$ ∂fS(w,t)∂t=−Π(w,t)fS(w,t)+QS(w,t), $
|
(2.1a) |
$ ∂fI(w,t)∂t=Π(w,t)fS(w,t)−rfI(w,t)+QI(w,t), $
|
(2.1b) |
$ ∂fR(w,t)∂t=rfI(w,t)+QR(w,t), $
|
(2.1c) |
where
$ QL(w,t)=∑J∈Ω1τLJQ(fL,fJ)(w,t),L∈Ω, $
|
is a combination of collision operators, and represents the interaction between heterogeneous agents [22,44]. The collision term $ Q(f_{L}, f_{J}) $ describes the changes in wealth distribution resulting from the microscopic interactions between individuals from groups $ L $ and $ J $. The relaxation parameters $ \tau_{LJ} $ ($ L, J\in\Omega $) before the collision operators depend on the behavior patterns of agents and the share of transactions [45], reflecting the impact of the infectious disease on the frequency of wealth exchange.
In (2.1), the time evolution of $ f_{L}(w, t) $ ($ L\in \Omega $) is expressed as the additive coupling of collision process and transport terms. The transport terms $ \Pi(w, t)f_{S}(w, t) $ and $ r f_{I}(w, t) $ consider the shift of agents from one category to another, while the change in health status is due to contact with infected persons or medical treatment. In (2.1b) and (2.1c), constant $ r > 0 $ is the recovery rate and $ 1/r $ represents the average infection period [11]. The spread of the epidemic is governed by the local incidence growth rate
$ Π(w,t)=∫R+ρ(w,v,t)fI(v,t)dv, $
|
where $ \rho(w, v, t) $ is the contact rate of two agents possessing wealth $ w $ and $ v $ at time $ t $.
Let $ L(t) = \int_{\mathbb{R}_{+}}f_{L}(w, t)dw $, $ L\in \Omega $ represents the proportion of agents in group $ L $. In this work, we take $ \rho(w, v, t) = \rho(t) > \rho_{0} > 0 $, where the constant $ \rho_{0} $ is a lower bound of $ \rho(t) $, then $ \Pi(w, t) = \rho(t)I(t) $.
In the background of an epidemic, we consider the binary wealth exchange model
$ w∗=w−[PL⋅H(I(t))+Φ(w¯W)]w+[PJ⋅H(I(t))+Φ(v¯W)]v+ηw, $
|
(2.2a) |
$ v∗=v−[PJ⋅H(I(t))+Φ(v¯W)]v+[PL⋅H(I(t))+Φ(w¯W)]w+ηv. $
|
(2.2b) |
In the wealth exchange rule (2.2), an agent with infection status $ L\in \Omega $ and wealth $ w $ interacts with another agent with infection status $ J\in \Omega $ and wealth $ v $, and their post-trade wealth is $ w^{*} $ and $ v^{*} $, respectively.
According to (2.2), the change of each agent's wealth in a single interaction comes from two mechanisms, the deterministic exchange part and the random fluctuation caused by market risks. The certain exchange part of each agent's wealth depends on the effect of infectious diseases and the impact of the individual's psychology on the transaction. In terms of a mathematical expression, the exchange propensity of the agent with wealth $ w $ and infection state $ L $ is represented by $ P_{L}\cdot H(I(t))+\Phi(\frac{w}{\overline{W}}) $. Specifically, $ P_{L} $ is the exchange propensity of agents with infection state $ L $ under normal circumstances, which is the same as that in the CPT model (1.1). $ H(I(t))\leq 1 $ is a non-increasing function of the infected fraction $ I(t) $ and is used to measure the impact of the epidemic on people's participation in economic activities. We take $ H(I(t)) = \frac{1}{1+I(t)} $. The value function $ \Phi(\frac{w}{\overline{W}}) $, which measures the psychological impact of an agent's wealth level on his trading decision, is an application of the prospect theory [28]. The constant $ \overline{W} $ represents a reference wealth level, such as average wealth. The uncertainty is represented by the random variable $ \eta $ with mean zero and variance $ \sigma $. To ensure that the post-trade wealth is nonnegative, we suppose that $ \eta\geq P_{L}\cdot H(I(t))+\Phi(\frac{w}{\overline{W}})-1 $. In addition, to guarantee that the kinetic equations (2.1) is well posed [13], we assume that
$ maxL∈{S,I,R}{[PL⋅H(I(t))+Φ(w¯W)]2+[1−PL⋅H(I(t))−Φ(w¯W)]2}+σ<1. $
|
(2.3) |
For the variable $ s\geq 0 $, we consider the value function
$ Φ(s)=μeϵ(s−1)−1νeϵ(s−1)+1,ν≥1, $
|
(2.4) |
where the constant $ \mu\in [0, 1) $ measures the influence of individual psychology on trading decision-making, and the constant $ 0 < \epsilon < 1 $ denotes the intensity of the frequency of wealth interaction. For fixed $ \mu $, $ \epsilon $ and $ s $, function $ |\Phi(s)| $ decreases with respect to parameter $ \nu $. (2.4) is a generalization of the value function $ \Phi_{1}^{\epsilon}(s) $ in [29],
$ Φϵ1(s)=μeϵ(s−1)−1eϵ(s−1)+1. $
|
Compared with $ \Phi_{1}^{\epsilon}(s) $, parameter $ \nu > 1 $ in (2.4) prevents the possibility that when the wealth is large, the proportion of increased investment is too high, and when the wealth is small, the conservative psychology leads to little investment. The images of function $ \Phi(s) $ with $ \mu = 0.1 $ and different values of parameters $ \nu $ and $ \epsilon $ are presented in Figure 1.
The Eq (2.4) satisfies the properties of the value function in [28], which are described as follows:
1) $ \Phi(s) $ monotonically increases with $ s $ and has finite upper and lower bounds
$ lims→+∞Φ(s)=μν≥Φ(s)≥lims→0Φ(s)=μν[1−1+ννe−ϵ+1]<0. $
|
(2.5) |
2) $ s = 1 $ is the reference point, i.e., $ \Phi(s = 1) = 0 $.
3) In the case of $ \epsilon < \log \nu $, we have $ [\Phi(s)]^{''} < 0 $ for every $ s\geq 0 $. Namely, $ \Phi(s) $ is a concave function on $ \mathbb{R}_{+} $. In the case of $ \log \nu < \epsilon < 1 $, $ s = 1-\frac{1}{\epsilon}\log \nu\in(0, 1) $ is the inflection point, and
$ [Φ(s)]″{>0,s<1−1ϵlogν,<0,s>1−1ϵlogν. $
|
4) Since $ \nu\geq1 $, we get
$ Φ(1+ζ)<−Φ(1−ζ),Φ′(1+ζ)<Φ′(1−ζ),ζ∈(0,1). $
|
Property 4) states that the value function (2.4) is asymmetric to the point $ s = 1 $, which can also be observed in Figure 1(b). It corresponds to a phenomenon that the decision-making behavior of agents possessing wealth higher than the reference level $ \overline{W} $ is more robust, namely, the fluctuation of decision-making affected by psychology is more gentle. Moreover, this property also indicates that agents with wealth under the reference level $ \overline{W} $ tend to save more wealth.
According to the boundedness (2.5), the parameters in the binary exchange rule (2.2) satisfy $ P_{L}+\mu/\nu < 1 $, $ \eta\geq P_{L}+\mu/\nu -1 $ and $ P_{L}+\frac{\mu}{\nu}(1-\frac{1+\nu}{\nu e^{-\epsilon}+1}) > 0 $ for every $ \epsilon\in(0, 1) $, $ L\in\Omega $. Since $ \frac{\mu}{\nu}(\frac{1+\nu}{\nu e^{-\epsilon}+1}-1) $ increases with $ \epsilon $, we have $ P_{L}\geq \mu\frac{e-1}{\nu+e} $, $ L\in\Omega $.
In (2.1), the Boltzmann-type collision operator $ Q(f_{L}, f_{L}) $ ($ L\in \Omega $) denotes the binary interaction of agents with the same infection state $ L $, and $ Q(f_{L}, f_{J}) $ with $ L\neq J $ ($ L, J\in \Omega $) represents the binary interaction of agents with different infection states $ L $ and $ J $. For any test function $ \psi(w)\in C_{0}^{\infty}(\mathbb{R}_{+}) $, we have [13]
$ ∫R+Q(fL,fJ)(w,t)ψ(w)dw=∫R2+⟨ψ(w∗)−ψ(w)⟩fL(w,t)fJ(v,t)dvdw, $
|
(3.1) |
where $ \langle\cdot\rangle $ is the expectation operator with respect to the random variable $ \eta $.
We take the relaxation time $ \tau_{LJ} = 1 $ ($ L, J\in\Omega $) in (2.1). Then, the weak form of the system (2.1) is
$ ddt∫R+fS(w,t)ψ(w)dw=−ρ(t)I(t)∫R+fS(w,t)ψ(w)dw+˜QS, $
|
(3.2a) |
$ ddt∫R+fI(w,t)ψ(w)dw=ρ(t)I(t)∫R+fS(w,t)ψ(w)dw−r∫R+fI(w,t)ψ(w)dw+˜QI, $
|
(3.2b) |
$ ddt∫R+fR(w,t)ψ(w)dw=r∫R+fI(w,t)ψ(w)dw+˜QR, $
|
(3.2c) |
where
$ ˜QL=∑J∈Ω∫R+Q(fL,fJ)(w,t)ψ(w)dw,L∈Ω. $
|
Substituting $ \psi(w) = 1 $ into (3.2), we obtain that the fractions of the three agent groups satisfy
$ dS(t)dt=−ρ(t)S(t)I(t), $
|
(3.3a) |
$ dI(t)dt=ρ(t)S(t)I(t)−rI(t), $
|
(3.3b) |
$ dR(t)dt=rI(t) $
|
(3.3c) |
and $ S(t)+I(t)+R(t)\equiv 1 $, which is due to the normalization condition of $ f(w, t) $. If $ \rho(t) = \rho $ is a constant, (3.3) is the classical SIR model, which is mathematically and epidemiologically well-posed [11]. In the SIR model, the contact number $ \gamma $, which refers to the average number of contacts of an infected person during the period of infection, is equal to the ratio of contact rate $ \rho(t) $ to recovery rate $ r $ (i.e. $ \gamma = \rho(t)/r $) [11]. Let $ \gamma_{0} = \rho/r $. For the classical SIR model (3.3), Hethcote [10] gives the properties of its solution, which is repeated as the following Theorem. Denote $ L^{\infty} = \lim\limits_{t\mapsto +\infty}L(t) $, $ f_{L}^{\infty}(w) = \lim\limits_{t\mapsto +\infty}f_{L}(w, t) $ with $ L\in \Omega. $
Theorem 3.1. ([10]) Let $ (S(t), I(t)) $ be a solution of the classical SIR model in
$ {(S(t),I(t))|S(t)≥0,I(t)≥0,S(t)+I(t)≤1}. $
|
If $ \gamma_{0}S_{0}\leq 1 $, then $ I(t) $ decreases to zero as $ t\mapsto \infty $. If $ \gamma_{0}S_{0} > 1 $, then $ I(t) $ increases to a maximum $ I_{max} = I_{0}+S_{0}-1/\gamma_{0}-[ln(\gamma_{0}S_{0})]/\gamma_{0} $ and then decreases to 0 as $ t\mapsto\infty $. The susceptible proportion $ S(t) $ is a decreasing function and $ S^{\infty}\in (0, 1/\gamma_{0}) $ is the unique root of the equation
$ S0+I0−S∞+1γ0lnS∞S0=0. $
|
(3.4) |
Theorem 3.1 implies that the infected fraction $ I(t) $ tends to zero in the steady-state (i.e., $ I^{\infty} = 0 $). Combined with the non-negativity of the distribution function, the wealth distribution of the infected population in the steady-state is zero, i.e., $ f_{I}^{\infty}(w) = 0 $. Then, in the steady-state, the order moments of wealth distribution of the infected population are zero. When $ I^{\infty} = 0 $, we have $ \lim\limits_{t\mapsto+\infty}\Pi(w, t) = 0 $.
Let $ m_{L}(t) = \int_{\mathbb{R}_{+}}wf_{L}(w, t)dw $ ($ L\in \Omega $) represent the mean wealth of group $ L $. According to (2.2), the whole agent system's wealth is conserved in the mean, i.e., $ \langle w^{*}+ v^{*}\rangle = w+ v $, then $ \sum\limits_{L\in\Omega} m_{L}(t): = m $, $ t\geq 0 $.
Let $ E_{L}(t) = \int_{\mathbb{R}_{+}} w^{2} f_{L}(w, t) dw $ ($ L\in\Omega $) represent the second moment of $ f_{L}(w, t) $. Denote $ m_{L}^{\infty} = \lim\limits_{t\mapsto +\infty}m_{L}(t) $ and $ E_{L}^{\infty} = \lim\limits_{t\mapsto +\infty}E_{L}(t) $.
Taking $ \psi(w) = w $ and substituting (2.2) into (3.1) arise
$ ∫R+Q(fL,fJ)(w,t)wdw=−[PL⋅H(I(t))]⋅mL(t)⋅J(t)+[PJ⋅H(I(t))]⋅mJ(t)⋅L(t)−J(t)∫R+Φ(w¯W)wfL(w,t)dw+L(t)∫R+Φ(v¯W)vfJ(v,t)dv. $
|
Denote
$ FL(t):=∫R+Φ(v¯W)vfL(v,t)dv. $
|
We get
$ dmS(t)dt=−ρ(t)I(t)mS(t)+MS(t), $
|
(3.5a) |
$ dmI(t)dt=ρ(t)I(t)mS(t)−rmI(t)+MI(t), $
|
(3.5b) |
$ dmR(t)dt=rmI(t)+MR(t), $
|
(3.5c) |
where
$ ML(t)=H(I(t))⋅L(t)⋅∑J∈ΩPJmJ(t)−PL⋅H(I(t))mL(t)+L(t)∑J∈ΩFJ(t)−FL(t),L∈Ω. $
|
If $ I^{\infty} = 0 $, we have $ m_{I}^{\infty} = 0 $. Denote $ F_{J}^{\infty} = \lim\limits_{t\mapsto +\infty}F_{J}(t) $. From (3.5a) and (3.5c), the mean wealth $ m_{S}^{\infty} $ and $ m_{R}^{\infty} $ satisfy
$ S∞(PSm∞S+PRm∞R)−PSm∞S+S∞(F∞S+F∞R)−F∞S=0, $
|
(3.6a) |
$ R∞(PSm∞S+PRm∞R)−PRm∞R+R∞(F∞S+F∞R)−F∞R=0, $
|
(3.6b) |
where $ R^{\infty} = 1-S^{\infty} $ and $ m_{S}^{\infty}+m_{R}^{\infty} = m $. Since $ \Phi(\frac{v}{\overline{W}}) $ is a nonlinear function of variable $ v $, the mean wealth $ m_{S}^{\infty} $ and $ m_{R}^{\infty} $ cannot be solved explicitly from (3.6), but their upper and lower bounds can be obtained. The value function (2.4) has an upper and lower bound (2.5), so we have $ F_{S}^{\infty} \in\big[\mu \frac{e^{-\epsilon}-1}{\nu e^{-\epsilon}+1} m_{S}^{\infty}, \frac{\mu}{\nu}m_{S}^{\infty} \big] $ and $ F_{R}^{\infty} \in\big[\mu \frac{e^{-\epsilon}-1}{\nu e^{-\epsilon}+1} m_{R}^{\infty}, \frac{\mu}{\nu}m_{R}^{\infty} \big] $. Then we obtain
$ m∞S∈[PR+μe−ϵ−1νe−ϵ+1DRSS∞m,PR+μνDSRS∞m],m∞R∈[PS+μe−ϵ−1νe−ϵ+1DSRR∞m,PS+μνDRSR∞m], $
|
where $ D_{SR} = S^{\infty} (P_{R}+\frac{\mu}{\nu}) +R^{\infty} (P_{S}+\mu \frac{e^{-\epsilon}-1}{\nu e^{-\epsilon}+1}) $ and $ D_{RS} = S^{\infty} (P_{R}++\mu \frac{e^{-\epsilon}-1}{\nu e^{-\epsilon}+1}) + R^{\infty} (P_{S}+\frac{\mu}{\nu}) $.
The Boltzmann-like system (2.1) describes how wealth distribution evolves under the course of an epidemic, but its explicit solutions are difficult to obtain. Utilizing the quasi-invariant limit method [40], the Boltzmann-type equations are turned into the Fokker-Planck equations. We can use the stationary solution of the Fokker-Planck system to depict the large-time behavior of wealth distribution.
Letting $ 0 < \epsilon\ll 1 $ and employing the scaled quantities
$ PJ→ϵPJ,η→√ϵη,J∈Ω. $
|
(4.1) |
The binary wealth exchange model (2.2) becomes
$ w∗−w=−[ϵPL⋅H(I(t))+Φ(w¯W)]w+[ϵPJ⋅H(I(t))+Φ(v¯W)]v+√ϵηw, $
|
(4.2a) |
$ v∗−v=−[ϵPJ⋅H(I(t))+Φ(v¯W)]v+[ϵPL⋅H(I(t))+Φ(w¯W)]w+√ϵηv, $
|
(4.2b) |
where $ L, J\in\Omega $.
The scaled micro wealth exchange model (4.2) indicates that a single interaction arises only small changes in wealth. To preserve the effects of all parameters in the exchange rule (2.2) when taking the limit $ \epsilon\mapsto 0 $, the agents need to do much more interactions within a time period, that is, to increase the interaction frequency. Therefore, we take $ \tau_{LJ}\to\epsilon\tau_{LJ} $, which also makes the evolution of the distributions independent of the scaling parameter $ \epsilon $. Then, the weak form of the scaled system (2.1) reads
$ ddt∫R+fS(w,t)ψ(w)dw=−∫R+Π(w,t)fS(w,t)ψ(w)dw+˜QϵS, $
|
(4.3a) |
$ ddt∫R+fI(w,t)ψ(w)dw=∫R+Π(w,t)fS(w,t)ψ(w)dw−r∫R+fI(w,t)ψ(w)dw+˜QϵI, $
|
(4.3b) |
$ ddt∫R+fR(w,t)ψ(w)dw=r∫R+fI(w,t)ψ(w)dw+˜QϵR, $
|
(4.3c) |
where
$ ˜QϵL=∑J∈Ω1ϵτLJ∫R+Qϵ(fL,fJ)(w,t)ψ(w)dw,L∈Ω. $
|
Taking a Taylor expansion of $ \psi(w^{*}) $ around $ w $, and substituting the expansion into the collision terms on the right of (4.3), we obtain
$ 1ϵτLJ∫R+Qϵ(fL,fJ)(w,t)ψ(w)dw=1ϵτLJ∫R2+⟨ψ′(w)(w∗−w)+ψ″(w)2(w∗−w)2⟩fL(w,t)fJ(v,t)dvdw+RLJ(ϵ). $
|
(4.4) |
The residual $ R_{LJ}(\epsilon) $ describes the higher-order terms of $ \epsilon $. In fact, for any test function $ \psi(w) $ belonging to the second-order $ H\ddot{o}lder $ space $ \mathcal{C}_{0}^{2+\alpha}(R_{+}) $ with $ \alpha\in(0, 1) $, we have
$ RLJ(ϵ)=1ϵτLJ∫R2+⟨ψ″(˜w)−ψ″(w)2(w∗−w)2⟩fL(w,t)fJ(v,t)dvdw,=O(ϵ1+α), $
|
where $ \tilde{w} = \theta w^{*} + (1-\theta)w $ for some $ \theta \in (0, 1) $.
Using
$ limϵ↦01ϵΦ(s)=limϵ↦01ϵμeϵ(s−1)−1νeϵ(s−1)+1=μν+1(s−1)≥−μν+1, $
|
(4.5) |
the limit of (4.4) is expressed as
$ limϵ↦01ϵτLJ∫R+Qϵ(fL,fJ)(w,t)ψ(w)dw=1τLJ∫R+{−∂∂w[HLJ(w,t)fL(w,t)]+σ2∂2∂w2[J(t)⋅w2fL(w,t)]}ψ(w)dw, $
|
(4.6) |
where
$ HLJ(w,t)=−[PL⋅H(I(t))+μν+1(w¯W−1)]⋅w⋅J(t)+[PJ⋅H(I(t))−μν+1]⋅mJ(t)+μ(ν+1)¯WEJ(t). $
|
Substituting (4.4) into (4.3), setting $ \tau_{LJ} = 1 $ ($ L, J\in\Omega $), taking $ \epsilon\mapsto 0 $ and combining with (4.6), we obtain
$ ∂fS(w,t)∂t=−Π(w,t)fS(w,t)−∂∂w[BS(w,t)fS(w,t)]+12∂2∂w2[σw2fS(w,t)], $
|
(4.7a) |
$ ∂fI(w,t)∂t=Π(w,t)fS(w,t)−rfI(w,t)−∂∂w[BI(w,t)fI(w,t)]+12∂2∂w2[σw2fI(w,t)], $
|
(4.7b) |
$ ∂fR(w,t)∂t=rfI(w,t)−∂∂w[BR(w,t)fR(w,t)]+12∂2∂w2[σw2fR(w,t)], $
|
(4.7c) |
where
$ BL(w,t)=−[PL⋅H(I(t))+μν+1(w¯W−1)]⋅w+H(I(t))⋅∑J∈ΩPJ⋅mJ(t)−μν+1m+μ(ν+1)¯W∑J∈ΩEJ(t),L∈Ω. $
|
The boundary conditions are
$ BL(w,t)fL(w,t)|w=+∞w=0=0,∂∂w[w2fL(w,t)]|w=+∞w=0=0,t≥0,L∈Ω. $
|
The system (4.7) maintains the memory of the micro-dynamic (2.2) through relevant parameters $ \mu $, $ \nu $, $ \overline{W} $, $ \sigma $ and $ P_{J} $ ($ J\in\Omega $).
As a special case of explicitly finding the steady-state solution of (4.7), we suppose that the contact rate $ \rho(t) = \rho > 0 $ is a constant. From Theorem 3.1, there are $ I^{\infty} = 0 $, $ f_{I}^{\infty}(w) = 0 $ and $ S^{\infty} $ is the unique solution of (3.4). The mean wealth $ m_{S}^{\infty} $ and $ m_{R}^{\infty} $ are bounded and belong to $ [0, m] $.
Proposition 4.1. When the steady-state infected fraction $ I^{\infty} = 0 $, the moments of the stationary solutions of the Boltzmann-like system (4.3) satisfy the recursive relationship
$ μ(ν+1)¯WMn+1,∞L=((n−1)σ2+μν+1−PL)Mn,∞L+(∑J∈{S,R}PJm∞J−μmν+1+μ(ν+1)¯W∑J∈{S,R}E∞J)Mn−1,∞L, $
|
(4.8) |
where
$ Mn,∞L=∫R+wnf∞L(w)dw,L∈{S,R},n=1,2,3,..., $
|
denotes the $ n $-order moment of the stationary wealth distribution of agents with infection state $ L $.
Proof. When $ I^{\infty} = 0 $, we have $ f_{I}^{\infty}(w) = 0 $ and $ M_{I}^{n, \infty} = 0 $.
For the case of $ n = 1 $. Substituting $ \psi(w) = w $ into (4.3), and using the scaled exchange rule (4.2), then the steady-state mean wealth $ m_{L}^{\infty} $ and energy $ E_{L}^{\infty} $ satisfy
$ (μν+1−PL)m∞L−μ(ν+1)L∞m+L∞∑J∈{S,R}PJ⋅m∞J=μ(ν+1)¯W(E∞L−L∞∑J∈{S,R}E∞J), $
|
(4.9) |
which is consistent with (4.8) when $ n = 1 $.
When $ n\geq 2 $, substituting $ \psi(w) = w^{n} $ into (4.3) and (4.4), letting $ \epsilon\mapsto 0 $ and using (4.5), we have
$ ∑J∈{S,R}∫R2+{−[PL+μν+1(w¯W−1)]w+[PJ+μν+1(v¯W−1)]v}wn−1f∞L(w)f∞J(v)dvdw+(n−1)σ2∑J∈{S,R}∫R2+wnf∞L(w)f∞J(v)dvdw=0. $
|
(4.10) |
Then the recursive relation (4.8) is deduced through a simple calculation of (4.10).
In the scaling (4.1), condition (2.3) for $ \epsilon\mapsto 0 $ becomes
$ minL∈{S,I,R}[PL+limϵ↦01ϵΦ(s)]>σ2. $
|
(4.11) |
Using the inequality in (4.5), a sufficient condition for (4.11) is $ P_{L}-\frac{\mu}{\nu+1}-\frac{\sigma}{2} > 0 $ with $ L\in \{S, I, R\} $. Employing the H$ \ddot{o} $lder's inequality, we have
$ (m∞L)2L∞≤E∞L,(E∞L)2m∞L≤M3,∞L,L∈{S,R}. $
|
(4.12) |
Let $ n = 2 $ in (4.8). Using (4.9) and (4.12), $ E_{L}^{\infty} $ has an upper bound
$ E∞L≤PL−μν+1PL−σ2−μν+1⋅(m∞L)2L∞,L∈{S,R}. $
|
(4.13) |
In the case of $ \mu\neq 0 $, according to the recursive formula (4.8) and the boundedness of the first two moments of wealth distributions (see (4.12) and (4.13)), we can obtain the boundedness of higher-order moments of wealth distributions in the stationary situation.
From (4.7), the stationary distribution $ f_{L}^{\infty}(w) $ ($ L\in \{S, R\} $) satisfies the ordinary differential equation
$ (bw2−aLw−cL)f∞L(w)+σ2ddw[w2f∞L(w)]=0, $
|
where
$ aL=μν+1−PL≤0,b=μ(ν+1)¯W≥0,cL=μ(ν+1)¯W⋅E∞LL∞+(PL−μν+1)⋅m∞LL∞>0. $
|
From (4.9), we have
$ cL=μ(ν+1)¯W⋅E∞LL∞+(PL−μν+1)⋅m∞LL∞=∑J∈{S,I,R}PJ⋅m∞J−μν+1m+μ(ν+1)¯W∑J∈{S,I,R}E∞J:=c, $
|
which is independent of the subscript $ L $. Then the stationary wealth distribution of the susceptible (recovered) group is expressed as
$ f∞L(w)=L∞CLw2(aL−σ)σe−2cσ1w−2bσw,L∈{S,R}, $
|
(4.14) |
where $ C_{L} $ is a regularization parameter such that $ \int_{\mathbb{R}_{+}}f_{L}^{\infty}(w)dw = L^{\infty} $. Note that
$ df∞L(w)dw=−2σ⋅1w2f∞L(w)⋅[bw2+(σ−aL)w−c]. $
|
Then, $ f_{L}^{\infty}(w) $ is a concave function in $ (0, +\infty) $ and reaches the maximum value at $ \tilde{w}_{L} = c/(\sigma-a_{L}) $ in the case of $ \mu = 0 $. When $ \mu\neq0 $, $ f_{L}^{\infty}(w) $ gets the maximum value at $ \tilde{w}_{L} = \big[(a_{L}-\sigma)+\sqrt{(a_{L}-\sigma)^{2}+4bc}\big]/(2b) $.
The whole stationary wealth distribution is given by
$ f∞(w)=S∞CSw2(aS−σ)σe−2cσ1w−2bσw+R∞CRw2(aR−σ)σe−2cσ1w−2bσw. $
|
(4.15) |
Proposition 4.2. The combination (4.15) of two unimodal distributions (4.14) is a unimodal or bimodal distribution.
Proof. Let
$ Υ(w)=∑L∈{S,R}f∞L(w)⋅[bw2+(σ−aL)w−c]. $
|
Then
$ df∞(w)dw=−2σ⋅1w2Υ(w). $
|
Using $ \lim\limits_{w\mapsto 0^{+}}\Upsilon(w) < 0 $ and $ \lim\limits_{w\mapsto +\infty}\Upsilon(w) > 0 $, thus the function $ \Upsilon(w) $ has at least one positive root, which corresponds to the maximum point of $ f^{\infty}(w) $. Taking the derivative of $ \Upsilon(w) $ with respect to $ w $, we get
$ dΥ(w)dw=−2σ1w2∑L∈{S,R}f∞L(w)⋅ϕL(w), $
|
where
$ ϕL(w)=b2w4+b(σ−2aL)w3+[(σ2−aL)(σ−aL)−2bc]w2−2c(σ−aL)w+c2. $
|
(4.16) |
Since $ f_{S}^{\infty}(w)\geq 0 $, $ f_{R}^{\infty}(w)\geq 0 $ and the number of variations in the sign of the polynomial $ \phi_{L}(w) $ is 2. Using the Descartes' rule of sign, the number of positive roots of $ d \Upsilon(w)/d w $ is 0 or 2. When $ d \Upsilon(w)/d w $ has no positive root, the function $ \Upsilon(w) $ has only on positive root. When $ d \Upsilon(w)/d w $ has two positive roots, the function $ \Upsilon(w) $ has three positive roots, corresponding to two maximum points and one minimum point of $ f^{\infty}(w) $, respectively.
The distribution (4.14) is the product of an inverse-gamma function and an exponential function, which is equivalent to
$ f∞L(w)=~CL⋅gL(w;aL,c,σ)⋅h(w;b,σ), $
|
(4.17) |
where
$ ~CL=L∞CL⋅σ2b⋅Γ(1−2aLσ)⋅(2cσ)2aLσ−1,gL(w;aL,c,σ)=1Γ(1−2aLσ)(2cσ)1−2aLσ⋅w2aLσ−2⋅e−2cσ⋅1w,h(w;b,σ)=2bσe−2bσw $
|
and $ \Gamma(\cdot) $ is the Gamma function. Note that $ g_{L}(w; a_{L}, c, \sigma) $ is the density of the inverse-gamma distribution $ IG_{L}(1-\frac{2a_{L}}{\sigma}, \frac{2c}{\sigma}) $, and $ h(w; b, \sigma) $ is the density of the exponential distribution $ E(\frac{2b}{\sigma}) $.
It is fascinating to note that the appearance of the exponential function part (i.e., $ h(w; b, \sigma) $) is mainly due to the function $ \Phi(\cdot) $ in (2.2). When the psychological factors are not considered in the wealth exchange (i.e., $ \mu = 0 $), (4.14) becomes an inverse-gamma function, which is the same as the stationary wealth distribution in [13], showing polynomial decay when $ w\mapsto\infty $. The decomposition form (4.17) suggests that, due to the exponential function $ h(w; b, \sigma) $, the tail of the wealth distribution (4.15) is slimmer than that of the inverse gamma distribution curve under appropriate parameters, and corresponds to a society with fewer super-rich people.
Under the framework of the SIR epidemic dynamic, by coupling the binary wealth exchange rule (2.2) with the Boltzmann-type dynamic system (2.1), we obtain the stationary wealth distribution (4.15), which is impacted by the epidemic-related parameters and the value function (2.4). In this part, we introduce the government contact control measures and vaccination into the kinetic system, and analyze the impact of different measures on epidemic dynamics and wealth distribution. Finally, in a fixed epidemic background, we explore the influence of the value function (2.4) on the stationary wealth distribution, and verify the possible situation that the whole stationary wealth distribution exists double peaks.
The Gini coefficient takes value in $ [0, 1] $ and measures wealth inequality. A larger Gini coefficient indicates a more unequal wealth distribution. The Gini coefficient is equal to the ratio of the area between the equality line and the Lorentz curve to the area below the equality line. Define the Lorenz curve as [40]
$ L(F(w))=∫w0vf∞(v)dv,F(w)=∫w0f∞(v)dv, $
|
then the Gini coefficient is
$ Gini=1−2∫10L(F(w))dF(w). $
|
In the following simulations, if there is no special declaration, we always take $ \rho = 0.3 $, $ I_{0} = 0.01 $, $ S_{0} = 0.99 $, $ P_{S} = 0.075 $, $ P_{R} = 0.15 $, $ m = 1 $, $ \sigma = 0.1 $, $ \overline{W} = 1 $ and $ \nu = 3 $.
Considering that the government takes non-drug containment measures to control the spread of infectious diseases. We take $ \rho(t) = \rho-\kappa I(t) > 0 $, where the constant $ \kappa \in [0, \min\{\rho/I(t)\}) $ measures the strength of contact control. Then, (3.3) is rewritten as
$ dS(t)dt=−[ρ−κI(t)]S(t)I(t), $
|
(5.1a) |
$ dI(t)dt=[ρ−κI(t)]S(t)I(t)−rI(t), $
|
(5.1b) |
$ dR(t)dt=rI(t). $
|
(5.1c) |
System (5.1) is an example of the implicit behavioral SIR model [46], and the contact rate $ \rho(t) $ decreases with the infected fraction $ I(t) $. When $ \kappa = 0 $, (5.1) becomes the classical SIR model in [10]. When $ \kappa\neq0 $, compared with the classical SIR model, a nonlinear term $ \kappa S(t)I^{2}(t) $ appears in (5.1). The contact number $ \gamma_{1} = [\rho-\kappa I(t)]/r\leq \rho/r $ varies with the infected fraction $ I(t) $. Because of $ 0 < \rho-\kappa I(t)\leq \rho $, $ S(t)\geq 0 $ is monotonically decreasing. Similar to Theorem 3.1, the infected fraction in the steady-state is zero, i.e., $ I^{\infty} = 0 $, as verified in Figure 2(a). Then, the wealth distribution of the infected group is zero in the steady-state, and the whole wealth distribution is still in the form of (4.15), in which $ S^{\infty} $ and $ R^{\infty} $ are the stationary solutions of (5.1).
Figure 2 depicts the epidemic dynamics and the wealth distribution with different values of parameters $ \kappa $ and $ r $. We take $ \mu = 0 $. The parameter value $ \rho = 0.3 $ and $ r = 0.04 $ are close to the epidemiological parameters estimated in [14], which are fitted from the COVID-19 data before the lockdown in three European countries (France, Italy and Spain). Figure 2(a) shows that with the increase of the recover rate $ r $ or the contact control strength $ \kappa $, the peak value of the infected fraction becomes smaller, and more agents keep the uninfected state. In particular, when $ r = 0.25 $, more than half of the susceptible people have the opportunity to remain uninfected during the epidemic.
The Gini coefficients of the wealth distributions in Figure 2(b) are $ Gini(r = 0.04, \kappa = 0) = 0.3127 $, $ Gini(r = 0.15, \kappa = 0) = 0.3739 $, $ Gini(r = 0.25, \kappa = 0) = 0.4257 $, $ Gini(r = 0.15, \kappa = 0.5) = 0.3901 $ and $ Gini(r = 0.15, \kappa = 1) = 0.3989 $. In the epidemic backgrounds in Figure 2(a), the wealth distribution curves and Lorentz curves in Figure 2(b) and their corresponding Gini coefficients show that the increase in $ r $ or $ \kappa $ leads the wealth distribution curve shifts to the left, and aggravates the wealth inequality. Combined with Figure 2(a), a reasonable explanation for this phenomenon is that when the contact number $ \gamma_{1} $ is small, the susceptible agents in the steady-state take a large proportion. Affected by the conservative exchange psychology of susceptible agents, that is, the exchange tendency of susceptible individuals is less than the tendency of recovered agents, the inequality of wealth distribution becomes worse.
One possible way to deal with the negative effect in Section 5.1.1 is vaccination. Through vaccination, the susceptible agent transforms directly into the recovered person. Compared with (2.1), we introduce a term $ \Xi(w, t)f_{S}(w, t) $ to represent the transfer of agents from the susceptible group to the recovered group due to vaccination. The Boltzmann-type system (2.1) is rewritten as
$ ∂fS(w,t)∂t=−Π(w,t)fS(w,t)−Ξ(w,t)fS(w,t)+QS(w,t), $
|
(5.2a) |
$ ∂fI(w,t)∂t=Π(w,t)fS(w,t)−rfI(w,t)+QI(w,t), $
|
(5.2b) |
$ ∂fR(w,t)∂t=rfI(w,t)+Ξ(w,t)fS(w,t)+QR(w,t), $
|
(5.2c) |
in which we take the transfer rate $ \Xi(w, t) = \int_{\mathbb{R}_{+}}\theta(t)f_{I}(v, t)dv $. To explore the effect of vaccination on the wealth distribution, we take the contact rate $ \rho(t) = \rho $ as a constant and $ \theta(t) = \beta \cdot \Psi_{\{t\geq T_{0}\}} $. The constant $ \beta > 0 $ represents the vaccination rate, $ T_{0} $ is the time of starting vaccination and $ \Psi_{\{t\geq T_{0}\}} $ denotes the indicator function on $ \{t|t\geq T_{0}\} $. Integrating the Eqs (5.2) over $ \mathbb{R}_{+} $ yields
$ dS(t)dt=−[ρ+θ(t)]S(t)I(t), $
|
(5.3a) |
$ dI(t)dt=ρS(t)I(t)−rI(t), $
|
(5.3b) |
$ dR(t)dt=[r+θ(t)S(t)]I(t). $
|
(5.3c) |
Since $ \theta(t)\geq 0 $, the consideration of vaccination does not affect the steady-state infected fraction $ I^{\infty} = 0 $, which is verified in Figure 3(a). Therefore, the stationary solution obtained from (5.2) has the same form as (4.15), while the susceptible and recovered fractions $ S^{\infty} $ and $ R^{\infty} $ are calculated from (5.3).
The impact of vaccination on epidemic and wealth distribution is shown in Figure 3, in which we take $ T_{0} = 30 $, $ r = 0.15 $ and $ \mu = 0 $. Figure 3 indicates that as the vaccination rate $ \beta $ increases, more agents become recovered. The total number of infected patients declines, and the distribution of wealth shifts to the right, reflecting an improvement in wealth inequality. The Gini coefficients of the wealth distributions in Figure 3(b) are $ Gini(\beta = 0) = 0.3739 $, $ Gini(\beta = 0.3) = 0.3551 $ and $ Gini(\beta = 0.7) = 0.3395 $. Therefore, according to the simulations in Figure 3, we infer that vaccination plays a role in preventing and controlling the epidemic and improving the economy. This change can not only effectively control the spread of the epidemic and reduce the infected fraction, but also have a positive impact on reducing wealth inequality.
In the exchange mechanism (2.2), the exchange propensity of agents is affected by their psychology, which is measured by the value function (2.4). The stationary wealth distribution of susceptible (infected) populations is obtained in the form of (4.14), which is the product of an inverse-gamma function and an exponential function (see (4.17)). It is different from the inverse-gamma steady-state distribution obtained from the usual socio-economic dynamic models [6,13].
In Figure 4, we compare the wealth distribution $ f^{\infty}(w) $ with different $ \mu $. Moreover, to verify the existence of bimodal distribution, we simulate the distribution $ f^{\infty}(w) $ with different values of the risk parameter $ \sigma $. We take $ P_{R} = 0.2 $ and choose the epidemic background corresponding to a recovery rate $ r = 0.25 $. The Gini coefficients of the wealth distributions in Figure 4(a) are $ Gini(\mu = 0) = 0.4476 $, $ Gini(\mu = 0.001) = 0.4444 $, $ Gini(\mu = 0.01) = 0.4258 $, $ Gini(\mu = 0.05) = 0.3856 $ and $ Gini(\mu = 0.08) = 0.3654 $. The Gini coefficients of the wealth distributions in Figure 4(b) are $ Gini(\mu = 0) = 0.2989 $, $ Gini(\mu = 0.001) = 0.2982 $, $ Gini(\mu = 0.01) = 0.2923 $, $ Gini(\mu = 0.05) = 0.2712 $ and $ Gini(\mu = 0.08) = 0.2591 $.
Figure 4 indicates that with the increase of psychological influence (i.e., the increase of $ \mu $), more agents are at the middle wealth level, the number of agents of both low and high wealth levels has declined. The Gini coefficient of different risk parameter values decreases with the increase of parameter $ \mu $. It also verifies the improvement of wealth inequality. Additionally, Figure 4(b) illustrates that the wealth distribution's tail becomes slimmer with the increase of $ \mu $, which corresponds to a society with fewer super-rich people. From the perspective of mathematical analysis, the larger the value of $ \mu $, the smaller the shape parameter $ 1-2a_{L}/\sigma $ of the inverse-gamma function $ g_{L}(w; a_{L}, c, \sigma) $. This corresponds to a fatter tail. Therefore, the existence of exponential function $ h(w; b, \sigma) $ plays a key role in thinning the tail.
Real economic behavior is complex and is affected by various factors, such as the market environment and the psychology of traders. Under the background of an epidemic, we utilizes the method of mixed rarefied gas dynamics to investigate the distribution of wealth. In the wealth exchange mechanism, the impact of agents' psychology on trading decisions is expressed by a value function, which satisfies the properties of the prospect theory [28]. Under the SIR epidemic, we obtain that the steady-state wealth distribution of the susceptible (recovered) group is the product of an inverse-gamma function and an exponential function, showing a single peak. The emergence of the exponential function part is caused by the nonlinear utility function (2.4). We prove and numerically verify that the wealth distribution of the whole agent system presents a bimodal distribution with appropriate parameters.
An increase in the recovery rate or the strength of contact control helps to curb the epidemic, keeping more people uninfected and reducing the peak value of the infected fraction. However, these measures are imperfect and may lead to economic recession, because the conservative trading psychology of susceptible agents caused by the fear of the virus is not changed. Vaccination, which transfers the susceptible person directly into a recovered individual, is numerically verified to have a positive impact on curbing epidemics and reducing wealth inequality. In addition, numerical simulations state that, with the increase of the psychological effect of agents, the population in the middle wealth level is increased, the Gini coefficient is decreased, and the tail of the multi-agent system's wealth distribution becomes thinner. These results indicate a reduction in the super-rich and wealth inequality.
This research is supported by the National Natural Science Foundation of China (No. 11471263). The authors are very grateful to the reviewers for their valuable and meaningful comments of the paper.
The authors declare there is no conflict of interest.
[1] |
Ganser-Pornillos BK, Yeager M, Pornillos O (2012) Assembly and architecture of HIV. Adv Exp Med Biol 726: 441-465. doi: 10.1007/978-1-4614-0980-9_20
![]() |
[2] | Sundquist WI, Krausslich HG (2012) HIV-1 assembly, budding, and maturation. Cold Spring Harb Perspect Med 2: a006924. |
[3] | Borggren M, Jansson M (2015) The Evolution of HIV-1 Interactions with Coreceptors and Mannose C-Type Lectin Receptors. Prog Mol Biol Transl Sci 129C: 109-140. |
[4] |
Miyauchi K, Kim Y, Latinovic O, et al. (2009) HIV enters cells via endocytosis and dynamin-dependent fusion with endosomes. Cell 137: 433-444. doi: 10.1016/j.cell.2009.02.046
![]() |
[5] |
Moulard M, Decroly E (2000) Maturation of HIV envelope glycoprotein precursors by cellular endoproteases. Biochim Biophys Acta 1469: 121-132. doi: 10.1016/S0304-4157(00)00014-9
![]() |
[6] |
Low JT, Garcia-Miranda P, Mouzakis KD, et al. (2014) Structure and dynamics of the HIV-1 frameshift element RNA. Biochemistry 53: 4282-4291. doi: 10.1021/bi5004926
![]() |
[7] |
Stephenson JD, Li H, Kenyon JC, et al. (2013) Three-dimensional RNA structure of the major HIV-1 packaging signal region. Structure 21: 951-962. doi: 10.1016/j.str.2013.04.008
![]() |
[8] |
Watts JM, Dang KK, Gorelick RJ, et al. (2009) Architecture and secondary structure of an entire HIV-1 RNA genome. Nature 460: 711-716. doi: 10.1038/nature08237
![]() |
[9] | Swanstrom R, Wills JW (1997) Synthesis, Assembly, and Processing of Viral Proteins. In: Coffin JM HS, Varmus HE, editor. Retroviruses. Cold Spring Harbor, New York: Cold Spring Harbor Laboratory Press. |
[10] |
Checkley MA, Luttge BG, Freed EO (2011) HIV-1 envelope glycoprotein biosynthesis, trafficking, and incorporation. J Mol Biol 410: 582-608. doi: 10.1016/j.jmb.2011.04.042
![]() |
[11] |
Postler TS, Desrosiers RC (2013) The tale of the long tail: the cytoplasmic domain of HIV-1 gp41. J Virol 87: 2-15. doi: 10.1128/JVI.02053-12
![]() |
[12] |
Bharat TA, Davey NE, Ulbrich P, et al. (2012) Structure of the immature retroviral capsid at 8 A resolution by cryo-electron microscopy. Nature 487: 385-389. doi: 10.1038/nature11169
![]() |
[13] | Maldonado JO, Martin JL, Mueller JD, et al. (2014) New insights into retroviral Gag-Gag and Gag-membrane interactions. Front Microbiol 5: 302. |
[14] | Sonnino S, Prinetti A (2013) Membrane domains and the “lipid raft” concept. Curr Med Chem 20: 4-21. |
[15] |
Levin JG, Mitra M, Mascarenhas A, et al. (2010) Role of HIV-1 nucleocapsid protein in HIV-1 reverse transcription. RNA Biol 7: 754-774. doi: 10.4161/rna.7.6.14115
![]() |
[16] |
Jiang J, Aiken C (2007) Maturation-dependent human immunodeficiency virus type 1 particle fusion requires a carboxyl-terminal region of the gp41 cytoplasmic tail. J Virol 81: 9999-10008. doi: 10.1128/JVI.00592-07
![]() |
[17] |
Wyma DJ, Jiang J, Shi J, et al. (2004) Coupling of human immunodeficiency virus type 1 fusion to virion maturation: a novel role of the gp41 cytoplasmic tail. J Virol 78: 3429-3435. doi: 10.1128/JVI.78.7.3429-3435.2004
![]() |
[18] | Aiken C, Zhang P (2013) HIV-1 Maturation. In: Freed EO, editor. Advances in HIV-1 Assembly and Release: Springer. |
[19] |
Forshey BM, von Schwedler U, Sundquist WI, et al. (2002) Formation of a human immunodeficiency virus type 1 core of optimal stability is crucial for viral replication. J Virol 76: 5667-5677. doi: 10.1128/JVI.76.11.5667-5677.2002
![]() |
[20] |
Ganser-Pornillos BK, Yeager M, Sundquist WI (2008) The structural biology of HIV assembly. Curr Opin Struct Biol 18: 203-217. doi: 10.1016/j.sbi.2008.02.001
![]() |
[21] |
Bush DL, Vogt VM (2014) In Vitro Assembly of Retroviruses. Annual Reviews in Virology 1: 561-580. doi: 10.1146/annurev-virology-031413-085427
![]() |
[22] |
Briggs JA (2013) Structural biology in situ--the potential of subtomogram averaging. Curr Opin Struct Biol 23: 261-267. doi: 10.1016/j.sbi.2013.02.003
![]() |
[23] |
Merk A, Subramaniam S (2013) HIV-1 envelope glycoprotein structure. Curr Opin Struct Biol 23: 268-276. doi: 10.1016/j.sbi.2013.03.007
![]() |
[24] |
Briggs JA, Simon MN, Gross I, et al. (2004) The stoichiometry of Gag protein in HIV-1. Nat Struct Mol Biol 11: 672-675. doi: 10.1038/nsmb785
![]() |
[25] |
Butan C, Winkler DC, Heymann JB, et al. (2008) RSV capsid polymorphism correlates with polymerization efficiency and envelope glycoprotein content: implications that nucleation controls morphogenesis. J Mol Biol 376: 1168-1181. doi: 10.1016/j.jmb.2007.12.003
![]() |
[26] |
Fuller SD, Wilk T, Gowen BE, et al. (1997) Cryo-electron microscopy reveals ordered domains in the immature HIV-1 particle. Curr Biol 7: 729-738. doi: 10.1016/S0960-9822(06)00331-9
![]() |
[27] |
Kingston RL, Olson NH, Vogt VM (2001) The organization of mature Rous sarcoma virus as studied by cryoelectron microscopy. J Struct Biol 136: 67-80. doi: 10.1006/jsbi.2001.4423
![]() |
[28] |
Yeager M, Wilson-Kubalek EM, Weiner SG, et al. (1998) Supramolecular organization of immature and mature murine leukemia virus revealed by electron cryo-microscopy: implications for retroviral assembly mechanisms. Proc Natl Acad Sci U S A 95: 7299-7304. doi: 10.1073/pnas.95.13.7299
![]() |
[29] |
Carlson LA, Briggs JA, Glass B, et al. (2008) Three-dimensional analysis of budding sites and released virus suggests a revised model for HIV-1 morphogenesis. Cell Host Microbe 4: 592-599. doi: 10.1016/j.chom.2008.10.013
![]() |
[30] |
Briggs JA, Johnson MC, Simon MN, et al. (2006) Cryo-electron microscopy reveals conserved and divergent features of gag packing in immature particles of Rous sarcoma virus and human immunodeficiency virus. J Mol Biol 355: 157-168. doi: 10.1016/j.jmb.2005.10.025
![]() |
[31] |
Briggs JA, Riches JD, Glass B, et al. (2009) Structure and assembly of immature HIV. Proc Natl Acad Sci U S A 106: 11090-11095. doi: 10.1073/pnas.0903535106
![]() |
[32] |
Keller PW, Huang RK, England MR, et al. (2013) A two-pronged structural analysis of retroviral maturation indicates that core formation proceeds by a disassembly-reassembly pathway rather than a displacive transition. J Virol 87: 13655-13664. doi: 10.1128/JVI.01408-13
![]() |
[33] |
Wright ER, Schooler JB, Ding HJ, et al. (2007) Electron cryotomography of immature HIV-1 virions reveals the structure of the CA and SP1 Gag shells. EMBO J 26: 2218-2226. doi: 10.1038/sj.emboj.7601664
![]() |
[34] |
de Marco A, Davey NE, Ulbrich P, et al. (2010) Conserved and variable features of Gag structure and arrangement in immature retrovirus particles. J Virol 84: 11729-11736. doi: 10.1128/JVI.01423-10
![]() |
[35] |
Datta SA, Temeselew LG, Crist RM, et al. (2011) On the role of the SP1 domain in HIV-1 particle assembly: a molecular switch? J Virol 85: 4111-4121. doi: 10.1128/JVI.00006-11
![]() |
[36] |
Liang C, Hu J, Russell RS, et al. (2002) Characterization of a putative alpha-helix across the capsid-SP1 boundary that is critical for the multimerization of human immunodeficiency virus type 1 gag. J Virol 76: 11729-11737. doi: 10.1128/JVI.76.22.11729-11737.2002
![]() |
[37] |
Briggs JA, Wilk T, Welker R, et al. (2003) Structural organization of authentic, mature HIV-1 virions and cores. EMBO J 22: 1707-1715. doi: 10.1093/emboj/cdg143
![]() |
[38] |
Ganser BK, Li S, Klishko VY, et al. (1999) Assembly and analysis of conical models for the HIV-1 core. Science 283: 80-83. doi: 10.1126/science.283.5398.80
![]() |
[39] |
Heymann JB, Butan C, Winkler DC, et al. (2008) Irregular and Semi-Regular Polyhedral Models for Rous Sarcoma Virus Cores. Comput Math Methods Med 9: 197-210. doi: 10.1080/17486700802168106
![]() |
[40] |
Cao S, Maldonado JO, Grigsby IF, et al. (2015) Analysis of human T-cell leukemia virus type 1 particles using cryo-electron tomography. J Virol 89: 2430-2435. doi: 10.1128/JVI.02358-14
![]() |
[41] |
Li S, Hill CP, Sundquist WI, et al. (2000) Image reconstructions of helical assemblies of the HIV-1 CA protein. Nature 407: 409-413. doi: 10.1038/35030177
![]() |
[42] |
Thomas D, Schultz P, Steven AC, et al. (1994) Mass analysis of biological macromolecular complexes by STEM. Biol Cell 80: 181-192. doi: 10.1111/j.1768-322X.1994.tb00929.x
![]() |
[43] |
Fogarty KH, Zhang W, Grigsby IF, et al. (2011) New insights into HTLV-1 particle structure, assembly, and Gag-Gag interactions in living cells. Viruses 3: 770-793. doi: 10.3390/v3060770
![]() |
[44] |
Johnson J, Chen Y, Mueller JD (2010) Characterization of brightness and stoichiometry of bright particles by flow-fluorescence fluctuation spectroscopy. Biophys J 99: 3084-3092. doi: 10.1016/j.bpj.2010.08.057
![]() |
[45] |
Parker SD, Wall JS, Hunter E (2001) Analysis of Mason-Pfizer monkey virus Gag particles by scanning transmission electron microscopy. J Virol 75: 9543-9548. doi: 10.1128/JVI.75.19.9543-9548.2001
![]() |
[46] | Vogt VM, Simon MN (1999) Mass determination of rous sarcoma virus virions by scanning transmission electron microscopy. J Virol 73: 7050-7055. |
[47] |
Yu F, Joshi SM, Ma YM, et al. (2001) Characterization of Rous sarcoma virus Gag particles assembled in vitro. J Virol 75: 2753-2764. doi: 10.1128/JVI.75.6.2753-2764.2001
![]() |
[48] |
Chen Y, Wu B, Musier-Forsyth K, et al. (2009) Fluorescence fluctuation spectroscopy on viral-like particles reveals variable gag stoichiometry. Biophys J 96: 1961-1969. doi: 10.1016/j.bpj.2008.10.067
![]() |
[49] |
Grigsby IF, Zhang W, Johnson JL, et al. (2010) Biophysical analysis of HTLV-1 particles reveals novel insights into particle morphology and Gag stochiometry. Retrovirology 7: 75. doi: 10.1186/1742-4690-7-75
![]() |
[50] |
Lanman J, Lam TT, Emmett MR, et al. (2004) Key interactions in HIV-1 maturation identified by hydrogen-deuterium exchange. Nat Struct Mol Biol 11: 676-677. doi: 10.1038/nsmb790
![]() |
[51] |
Briggs JA, Watson BE, Gowen BE, et al. (2004) Cryoelectron microscopy of mouse mammary tumor virus. J Virol 78: 2606-2608. doi: 10.1128/JVI.78.5.2606-2608.2004
![]() |
[52] |
Forster F, Medalia O, Zauberman N, et al. (2005) Retrovirus envelope protein complex structure in situ studied by cryo-electron tomography. Proc Natl Acad Sci U S A 102: 4729-4734. doi: 10.1073/pnas.0409178102
![]() |
[53] |
Briggs JA, Grunewald K, Glass B, et al. (2006) The mechanism of HIV-1 core assembly: insights from three-dimensional reconstructions of authentic virions. Structure 14: 15-20. doi: 10.1016/j.str.2005.09.010
![]() |
[54] |
Hamann MV, Mullers E, Reh J, et al. (2014) The cooperative function of arginine residues in the Prototype Foamy Virus Gag C-terminus mediates viral and cellular RNA encapsidation. Retrovirology 11: 87. doi: 10.1186/s12977-014-0087-7
![]() |
[55] |
Roux KH, Taylor KA (2007) AIDS virus envelope spike structure. Curr Opin Struct Biol 17: 244-252. doi: 10.1016/j.sbi.2007.03.008
![]() |
[56] |
Liu J, Bartesaghi A, Borgnia MJ, et al. (2008) Molecular architecture of native HIV-1 gp120 trimers. Nature 455: 109-113. doi: 10.1038/nature07159
![]() |
[57] |
Zanetti G, Briggs JA, Grunewald K, et al. (2006) Cryo-electron tomographic structure of an immunodeficiency virus envelope complex in situ. PLoS Pathog 2: e83. doi: 10.1371/journal.ppat.0020083
![]() |
[58] |
Harris A, Borgnia MJ, Shi D, et al. (2011) Trimeric HIV-1 glycoprotein gp140 immunogens and native HIV-1 envelope glycoproteins display the same closed and open quaternary molecular architectures. Proc Natl Acad Sci U S A 108: 11440-11445. doi: 10.1073/pnas.1101414108
![]() |
[59] |
Meyerson JR, Tran EE, Kuybeda O, et al. (2013) Molecular structures of trimeric HIV-1 Env in complex with small antibody derivatives. Proc Natl Acad Sci U S A 110: 513-518. doi: 10.1073/pnas.1214810110
![]() |
[60] |
Tran EE, Borgnia MJ, Kuybeda O, et al. (2012) Structural mechanism of trimeric HIV-1 envelope glycoprotein activation. PLoS Pathog 8: e1002797. doi: 10.1371/journal.ppat.1002797
![]() |
[61] |
White TA, Bartesaghi A, Borgnia MJ, et al. (2011) Three-dimensional structures of soluble CD4-bound states of trimeric simian immunodeficiency virus envelope glycoproteins determined by using cryo-electron tomography. J Virol 85: 12114-12123. doi: 10.1128/JVI.05297-11
![]() |
[62] |
White TA, Bartesaghi A, Borgnia MJ, et al. (2010) Molecular architectures of trimeric SIV and HIV-1 envelope glycoproteins on intact viruses: strain-dependent variation in quaternary structure. PLoS Pathog 6: e1001249. doi: 10.1371/journal.ppat.1001249
![]() |
[63] |
Loving R, Wu SR, Sjoberg M, et al. (2012) Maturation cleavage of the murine leukemia virus Env precursor separates the transmembrane subunits to prime it for receptor triggering. Proc Natl Acad Sci U S A 109: 7735-7740. doi: 10.1073/pnas.1118125109
![]() |
[64] |
Sjoberg M, Wu SR, Loving R, et al. (2014) Furin cleavage of the Moloney murine leukemia virus Env precursor reorganizes the spike structure. Proc Natl Acad Sci U S A 111: 6034-6039. doi: 10.1073/pnas.1317972111
![]() |
[65] |
Wu SR, Sjoberg M, Wallin M, et al. (2008) Turning of the receptor-binding domains opens up the murine leukaemia virus Env for membrane fusion. EMBO J 27: 2799-2808. doi: 10.1038/emboj.2008.187
![]() |
[66] |
Zhu P, Liu J, Bess J, Jr., et al. (2006) Distribution and three-dimensional structure of AIDS virus envelope spikes. Nature 441: 847-852. doi: 10.1038/nature04817
![]() |
[67] | Schur FK, Hagen WJ, Rumlova M, et al. (2015) Structure of the immature HIV-1 capsid in intact virus particles at 8.8 A resolution. . Nature 517: 505-508. |
[68] |
Pettersen EF, Goddard TD, Huang CC, et al. (2004) UCSF Chimera--a visualization system for exploratory research and analysis. J Comput Chem 25: 1605-1612. doi: 10.1002/jcc.20084
![]() |
[69] |
Zhao G, Perilla JR, Yufenyuy EL, et al. (2013) Mature HIV-1 capsid structure by cryo-electron microscopy and all-atom molecular dynamics. Nature 497: 643-646. doi: 10.1038/nature12162
![]() |
[70] |
Cardone G, Purdy JG, Cheng N, et al. (2009) Visualization of a missing link in retrovirus capsid assembly. Nature 457: 694-698. doi: 10.1038/nature07724
![]() |
[71] |
Hyun JK, Radjainia M, Kingston RL, et al. (2010) Proton-driven assembly of the Rous Sarcoma virus capsid protein results in the formation of icosahedral particles. J Biol Chem 285: 15056-15064. doi: 10.1074/jbc.M110.108209
![]() |
[72] |
Ganser-Pornillos BK, Cheng A, Yeager M (2007) Structure of full-length HIV-1 CA: a model for the mature capsid lattice. Cell 131: 70-79. doi: 10.1016/j.cell.2007.08.018
![]() |
[73] |
Bartesaghi A, Merk A, Borgnia MJ, et al. (2013) Prefusion structure of trimeric HIV-1 envelope glycoprotein determined by cryo-electron microscopy. Nat Struct Mol Biol 20: 1352-1357. doi: 10.1038/nsmb.2711
![]() |
[74] |
Lyumkis D, Julien JP, de Val N, et al. (2013) Cryo-EM structure of a fully glycosylated soluble cleaved HIV-1 envelope trimer. Science 342: 1484-1490. doi: 10.1126/science.1245627
![]() |
[75] |
Bartesaghi A, Lecumberry F, Sapiro G, et al. (2012) Protein secondary structure determination by constrained single-particle cryo-electron tomography. Structure 20: 2003-2013. doi: 10.1016/j.str.2012.10.016
![]() |
[76] | Schur FK, Hagen WJ, de Marco A, et al. (2013) Determination of protein structure at 8.5A resolution using cryo-electron tomography and sub-tomogram averaging. . J Struct Biol 184: 394-400. |
[77] | Tang C, Ndassa Y, Summers MF (2002) Structure of the N-terminal 283-residue fragment of the immature HIV-1 Gag polyprotein. Nat Struct Biol 9: 537-543. |
[78] |
Pornillos O, Ganser-Pornillos BK, Kelly BN, et al. (2009) X-ray structures of the hexameric building block of the HIV capsid. Cell 137: 1282-1292. doi: 10.1016/j.cell.2009.04.063
![]() |
[79] |
Macek P, Chmelik J, Krizova I, et al. (2009) NMR structure of the N-terminal domain of capsid protein from the mason-pfizer monkey virus. J Mol Biol 392: 100-114. doi: 10.1016/j.jmb.2009.06.029
![]() |
[80] |
Bharat TA, Castillo Menendez LR, Hagen WJ, et al. (2014) Cryo-electron microscopy of tubular arrays of HIV-1 Gag resolves structures essential for immature virus assembly. Proc Natl Acad Sci U S A 111: 8233-8238. doi: 10.1073/pnas.1401455111
![]() |
[81] |
Mao Y, Wang L, Gu C, et al. (2012) Subunit organization of the membrane-bound HIV-1 envelope glycoprotein trimer. Nat Struct Mol Biol 19: 893-899. doi: 10.1038/nsmb.2351
![]() |
[82] |
Mao Y, Wang L, Gu C, et al. (2013) Molecular architecture of the uncleaved HIV-1 envelope glycoprotein trimer. Proc Natl Acad Sci U S A 110: 12438-12443. doi: 10.1073/pnas.1307382110
![]() |
[83] | Cohen J Structural biology. Is high-tech view of HIV too good to be true? Science 341: 443-444. |
[84] | Henderson R Avoiding the pitfalls of single particle cryo-electron microscopy: Einstein from noise. Proc Natl Acad Sci U S A 110: 18037-18041. |
[85] | Mao Y, Castillo-Menendez LR, Sodroski JG Reply to Subramaniam, van Heel, and Henderson: Validity of the cryo-electron microscopy structures of the HIV-1 envelope glycoprotein complex. Proc Natl Acad Sci U S A 110: E4178-4182. |
[86] |
Frank GA, Narayan K, Bess JW, Jr., et al. (2015) Maturation of the HIV-1 core by a non-diffusional phase transition. Nat Commun 6: 5854. doi: 10.1038/ncomms6854
![]() |
[87] | van Heel M Finding trimeric HIV-1 envelope glycoproteins in random noise. Proc Natl Acad Sci U S A 110: E4175-4177. |
[88] | Mammano F, Ohagen A, Hoglund S, et al. (1994) Role of the major homology region of human immunodeficiency virus type 1 in virion morphogenesis. J Virol 68: 4927-4936. |
[89] |
von Schwedler UK, Stray KM, Garrus JE, et al. (2003) Functional surfaces of the human immunodeficiency virus type 1 capsid protein. J Virol 77: 5439-5450. doi: 10.1128/JVI.77.9.5439-5450.2003
![]() |
[90] |
Chu HH, Chang YF, Wang CT (2006) Mutations in the alpha-helix directly C-terminal to the major homology region of human immunodeficiency virus type 1 capsid protein disrupt Gag multimerization and markedly impair virus particle production. J Biomed Sci 13: 645-656. doi: 10.1007/s11373-006-9094-6
![]() |
[91] |
Gamble TR, Yoo S, Vajdos FF, et al. (1997) Structure of the carboxyl-terminal dimerization domain of the HIV-1 capsid protein. Science 278: 849-853. doi: 10.1126/science.278.5339.849
![]() |
[92] |
Ono A, Ablan SD, Lockett SJ, et al. (2004) Phosphatidylinositol (4,5) bisphosphate regulates HIV-1 Gag targeting to the plasma membrane. Proc Natl Acad Sci U S A 101: 14889-14894. doi: 10.1073/pnas.0405596101
![]() |
[93] |
Saad JS, Miller J, Tai J, et al. (2006) Structural basis for targeting HIV-1 Gag proteins to the plasma membrane for virus assembly. Proc Natl Acad Sci U S A 103: 11364-11369. doi: 10.1073/pnas.0602818103
![]() |
[94] |
Fogarty KH, Chen Y, Grigsby IF, et al. (2011) Characterization of cytoplasmic Gag-gag interactions by dual-color z-scan fluorescence fluctuation spectroscopy. Biophys J 100: 1587-1595. doi: 10.1016/j.bpj.2011.02.008
![]() |
[95] |
Lindwasser OW, Resh MD (2004) Human immunodeficiency virus type 1 Gag contains a dileucine-like motif that regulates association with multivesicular bodies. J Virol 78: 6013-6023. doi: 10.1128/JVI.78.11.6013-6023.2004
![]() |
[96] |
Muriaux D, Mirro J, Harvin D, et al. (2001) RNA is a structural element in retrovirus particles. Proc Natl Acad Sci U S A 98: 5246-5251. doi: 10.1073/pnas.091000398
![]() |
[97] |
Poole E, Strappe P, Mok HP, et al. (2005) HIV-1 Gag-RNA interaction occurs at a perinuclear/centrosomal site; analysis by confocal microscopy and FRET. Traffic 6: 741-755. doi: 10.1111/j.1600-0854.2005.00312.x
![]() |
[98] |
Bailey GD, Hyun JK, Mitra AK, et al. (2012) A structural model for the generation of continuous curvature on the surface of a retroviral capsid. J Mol Biol 417: 212-223. doi: 10.1016/j.jmb.2012.01.014
![]() |
[99] |
Mortuza GB, Haire LF, Stevens A, et al. (2004) High-resolution structure of a retroviral capsid hexameric amino-terminal domain. Nature 431: 481-485. doi: 10.1038/nature02915
![]() |
[100] |
Byeon IJ, Meng X, Jung J, et al. (2009) Structural convergence between Cryo-EM and NMR reveals intersubunit interactions critical for HIV-1 capsid function. Cell 139: 780-790. doi: 10.1016/j.cell.2009.10.010
![]() |
[101] |
Yang H, Ji X, Zhao G, et al. (2012) Structural insight into HIV-1 capsid recognition by rhesus TRIM5alpha. Proc Natl Acad Sci U S A 109: 18372-18377. doi: 10.1073/pnas.1210903109
![]() |
[102] |
Zhao G, Ke D, Vu T, et al. (2011) Rhesus TRIM5alpha disrupts the HIV-1 capsid at the inter-hexamer interfaces. PLoS Pathog 7: e1002009. doi: 10.1371/journal.ppat.1002009
![]() |
[103] | Gres AT, Kirby KA, KewalRamani VN, et al. (2015) X-ray crystal structures of native HIV-1 capsid protein reveal conformational variability. Science. |
[104] |
Dalgleish AG, Beverley PC, Clapham PR, et al. (1984) The CD4 (T4) antigen is an essential component of the receptor for the AIDS retrovirus. Nature 312: 763-767. doi: 10.1038/312763a0
![]() |
[105] |
Trkola A, Dragic T, Arthos J, et al. (1996) CD4-dependent, antibody-sensitive interactions between HIV-1 and its co-receptor CCR-5. Nature 384: 184-187. doi: 10.1038/384184a0
![]() |
[106] |
Wyatt R, Sodroski J (1998) The HIV-1 envelope glycoproteins: fusogens, antigens, and immunogens. Science 280: 1884-1888. doi: 10.1126/science.280.5371.1884
![]() |
[107] |
Buzon V, Natrajan G, Schibli D, et al. (2010) Crystal structure of HIV-1 gp41 including both fusion peptide and membrane proximal external regions. PLoS Pathog 6: e1000880. doi: 10.1371/journal.ppat.1000880
![]() |
[108] |
Chan DC, Fass D, Berger JM, et al. (1997) Core structure of gp41 from the HIV envelope glycoprotein. Cell 89: 263-273. doi: 10.1016/S0092-8674(00)80205-6
![]() |
[109] |
Tan K, Liu J, Wang J, et al. (1997) Atomic structure of a thermostable subdomain of HIV-1 gp41. Proc Natl Acad Sci U S A 94: 12303-12308. doi: 10.1073/pnas.94.23.12303
![]() |
[110] |
Weissenhorn W, Dessen A, Harrison SC, et al. (1997) Atomic structure of the ectodomain from HIV-1 gp41. Nature 387: 426-430. doi: 10.1038/387426a0
![]() |
[111] |
Chen L, Kwon YD, Zhou T, et al. (2009) Structural basis of immune evasion at the site of CD4 attachment on HIV-1 gp120. Science 326: 1123-1127. doi: 10.1126/science.1175868
![]() |
[112] |
Huang CC, Lam SN, Acharya P, et al. (2007) Structures of the CCR5 N terminus and of a tyrosine-sulfated antibody with HIV-1 gp120 and CD4. Science 317: 1930-1934. doi: 10.1126/science.1145373
![]() |
[113] |
Huang CC, Tang M, Zhang MY, et al. (2005) Structure of a V3-containing HIV-1 gp120 core. Science 310: 1025-1028. doi: 10.1126/science.1118398
![]() |
[114] |
Huang J, Ofek G, Laub L, et al. (2012) Broad and potent neutralization of HIV-1 by a gp41-specific human antibody. Nature 491: 406-412. doi: 10.1038/nature11544
![]() |
[115] |
Kwong PD, Wyatt R, Robinson J, et al. (1998) Structure of an HIV gp120 envelope glycoprotein in complex with the CD4 receptor and a neutralizing human antibody. Nature 393: 648-659. doi: 10.1038/31405
![]() |
[116] |
McLellan JS, Pancera M, Carrico C, et al. (2011) Structure of HIV-1 gp120 V1/V2 domain with broadly neutralizing antibody PG9. Nature 480: 336-343. doi: 10.1038/nature10696
![]() |
[117] |
Pejchal R, Doores KJ, Walker LM, et al. (2011) A potent and broad neutralizing antibody recognizes and penetrates the HIV glycan shield. Science 334: 1097-1103. doi: 10.1126/science.1213256
![]() |
[118] |
Ward AB, Wilson IA (2015) Insights into the trimeric HIV-1 envelope glycoprotein structure. Trends Biochem Sci 40: 101-107. doi: 10.1016/j.tibs.2014.12.006
![]() |
[119] |
Zhou T, Georgiev I, Wu X, et al. (2010) Structural basis for broad and potent neutralization of HIV-1 by antibody VRC01. Science 329: 811-817. doi: 10.1126/science.1192819
![]() |
[120] |
Zhou T, Xu L, Dey B, et al. (2007) Structural definition of a conserved neutralization epitope on HIV-1 gp120. Nature 445: 732-737. doi: 10.1038/nature05580
![]() |
[121] |
Dutta M, Liu J, Roux KH, et al. (2014) Visualization of retroviral envelope spikes in complex with the V3 loop antibody 447-52D on intact viruses by cryo-electron tomography. J Virol 88: 12265-12275. doi: 10.1128/JVI.01596-14
![]() |
[122] |
Harris AK, Bartesaghi A, Milne JL, et al. (2013) HIV-1 envelope glycoprotein trimers display open quaternary conformation when bound to the gp41 membrane-proximal external-region-directed broadly neutralizing antibody Z13e1. J Virol 87: 7191-7196. doi: 10.1128/JVI.03284-12
![]() |
[123] |
Hu G, Liu J, Taylor KA, et al. (2011) Structural comparison of HIV-1 envelope spikes with and without the V1/V2 loop. J Virol 85: 2741-2750. doi: 10.1128/JVI.01612-10
![]() |
[124] |
Zhu P, Winkler H, Chertova E, et al. (2008) Cryoelectron tomography of HIV-1 envelope spikes: further evidence for tripod-like legs. PLoS Pathog 4: e1000203. doi: 10.1371/journal.ppat.1000203
![]() |
[125] |
Sanders RW, Vesanen M, Schuelke N, et al. (2002) Stabilization of the soluble, cleaved, trimeric form of the envelope glycoprotein complex of human immunodeficiency virus type 1. J Virol 76: 8875-8889. doi: 10.1128/JVI.76.17.8875-8889.2002
![]() |
[126] |
Earl LA, Lifson JD, Subramaniam S (2013) Catching HIV 'in the act' with 3D electron microscopy. Trends Microbiol 21: 397-404. doi: 10.1016/j.tim.2013.06.004
![]() |
[127] |
Lucic V, Rigort A, Baumeister W (2013) Cryo-electron tomography: the challenge of doing structural biology in situ. J Cell Biol 202: 407-419. doi: 10.1083/jcb.201304193
![]() |
[128] |
Jun S, Ke D, Debiec K, et al. (2011) Direct visualization of HIV-1 with correlative live-cell microscopy and cryo-electron tomography. Structure 19: 1573-1581. doi: 10.1016/j.str.2011.09.006
![]() |
[129] | Jun S, Zhao G, Ning J, et al. (2013) Correlative microscopy for 3D structural analysis of dynamic interactions. J Vis Exp. |
[130] |
Majorovits E, Nejmeddine M, Tanaka Y, et al. (2008) Human T-lymphotropic virus-1 visualized at the virological synapse by electron tomography. PLoS One 3: e2251. doi: 10.1371/journal.pone.0002251
![]() |
[131] |
Martin N, Welsch S, Jolly C, et al. (2010) Virological synapse-mediated spread of human immunodeficiency virus type 1 between T cells is sensitive to entry inhibition. J Virol 84: 3516-3527. doi: 10.1128/JVI.02651-09
![]() |
[132] |
Do T, Murphy G, Earl LA, et al. (2014) Three-dimensional imaging of HIV-1 virological synapses reveals membrane architectures involved in virus transmission. J Virol 88: 10327-10339. doi: 10.1128/JVI.00788-14
![]() |
[133] | Drobne D (2013) 3D imaging of cells and tissues by focused ion beam/scanning electron microscopy (FIB/SEM). Methods Mol Biol 950: 275-292. |
[134] |
Risco C, Castro IFd, Sanz-Sánchez L, et al. (2014) Three-Dimensional Imaging of Viral Infections. Annual Review of Virology 1: 453-473. doi: 10.1146/annurev-virology-031413-085351
![]() |
[135] |
Kukulski W, Schorb M, Welsch S, et al. (2011) Correlated fluorescence and 3D electron microscopy with high sensitivity and spatial precision. J Cell Biol 192: 111-119. doi: 10.1083/jcb.201009037
![]() |
[136] |
Sartori A, Gatz R, Beck F, et al. (2007) Correlative microscopy: bridging the gap between fluorescence light microscopy and cryo-electron tomography. J Struct Biol 160: 135-145. doi: 10.1016/j.jsb.2007.07.011
![]() |
[137] |
Zhang P (2013) Correlative cryo-electron tomography and optical microscopy of cells. Curr Opin Struct Biol 23: 763-770. doi: 10.1016/j.sbi.2013.07.017
![]() |
[138] | Woodward CL, Cheng SN, Jensen GJ (2014) Electron cryo-tomography studies of maturing HIV-1 particles reveal the assembly pathway of the viral core. J Virol. |
[139] |
Carlson LA, de Marco A, Oberwinkler H, et al. (2010) Cryo electron tomography of native HIV-1 budding sites. PLoS Pathog 6: e1001173. doi: 10.1371/journal.ppat.1001173
![]() |
[140] |
Dobro MJ, Samson RY, Yu Z, et al. (2013) Electron cryotomography of ESCRT assemblies and dividing Sulfolobus cells suggests that spiraling filaments are involved in membrane scission. Mol Biol Cell 24: 2319-2327. doi: 10.1091/mbc.E12-11-0785
![]() |
[141] |
Effantin G, Dordor A, Sandrin V, et al. (2013) ESCRT-III CHMP2A and CHMP3 form variable helical polymers in vitro and act synergistically during HIV-1 budding. Cell Microbiol 15: 213-226. doi: 10.1111/cmi.12041
![]() |
[142] |
Shen QT, Schuh AL, Zheng Y, et al. (2014) Structural analysis and modeling reveals new mechanisms governing ESCRT-III spiral filament assembly. J Cell Biol 206: 763-777. doi: 10.1083/jcb.201403108
![]() |
[143] |
Van Engelenburg SB, Shtengel G, Sengupta P, et al. (2014) Distribution of ESCRT machinery at HIV assembly sites reveals virus scaffolding of ESCRT subunits. Science 343: 653-656. doi: 10.1126/science.1247786
![]() |
[144] |
de Marco A, Muller B, Glass B, et al. (2010) Structural analysis of HIV-1 maturation using cryo-electron tomography. PLoS Pathog 6: e1001215. doi: 10.1371/journal.ppat.1001215
![]() |
[145] |
de Marco A, Heuser AM, Glass B, et al. (2012) Role of the SP2 domain and its proteolytic cleavage in HIV-1 structural maturation and infectivity. J Virol 86: 13708-13716. doi: 10.1128/JVI.01704-12
![]() |
[146] |
Konnyu B, Sadiq SK, Turanyi T, et al. (2013) Gag-Pol processing during HIV-1 virion maturation: a systems biology approach. PLoS Comput Biol 9: e1003103. doi: 10.1371/journal.pcbi.1003103
![]() |
[147] |
Keller PW, Adamson CS, Heymann JB, et al. (2011) HIV-1 maturation inhibitor bevirimat stabilizes the immature Gag lattice. J Virol 85: 1420-1428. doi: 10.1128/JVI.01926-10
![]() |
[148] |
Benjamin J, Ganser-Pornillos BK, Tivol WF, et al. (2005) Three-dimensional structure of HIV-1 virus-like particles by electron cryotomography. J Mol Biol 346: 577-588. doi: 10.1016/j.jmb.2004.11.064
![]() |
[149] |
Levandovsky A, Zandi R (2009) Nonequilibirum assembly, retroviruses, and conical structures. Phys Rev Lett 102: 198102. doi: 10.1103/PhysRevLett.102.198102
![]() |
[150] |
Yu Z, Dobro MJ, Woodward CL, et al. (2013) Unclosed HIV-1 capsids suggest a curled sheet model of assembly. J Mol Biol 425: 112-123. doi: 10.1016/j.jmb.2012.10.006
![]() |
[151] |
Sougrat R, Bartesaghi A, Lifson JD, et al. (2007) Electron tomography of the contact between T cells and SIV/HIV-1: implications for viral entry. PLoS Pathog 3: e63. doi: 10.1371/journal.ppat.0030063
![]() |
[152] |
Cardone G, Brecher M, Fontana J, et al. (2012) Visualization of the two-step fusion process of the retrovirus avian sarcoma/leukosis virus by cryo-electron tomography. J Virol 86: 12129-12137. doi: 10.1128/JVI.01880-12
![]() |
[153] |
Felts RL, Narayan K, Estes JD, et al. (2010) 3D visualization of HIV transfer at the virological synapse between dendritic cells and T cells. Proc Natl Acad Sci U S A 107: 13336-13341. doi: 10.1073/pnas.1003040107
![]() |
[154] |
Sherer NM, Lehmann MJ, Jimenez-Soto LF, et al. (2007) Retroviruses can establish filopodial bridges for efficient cell-to-cell transmission. Nat Cell Biol 9: 310-315. doi: 10.1038/ncb1544
![]() |
[155] | Pique C, Jones KS (2012) Pathways of cell-cell transmission of HTLV-1. Front Microbiol 3: 378. |
[156] |
Hill CP, Worthylake D, Bancroft DP, et al. (1996) Crystal structures of the trimeric human immunodeficiency virus type 1 matrix protein: implications for membrane association and assembly. Proc Natl Acad Sci U S A 93: 3099-3104. doi: 10.1073/pnas.93.7.3099
![]() |
[157] |
Rao Z, Belyaev AS, Fry E, et al. (1995) Crystal structure of SIV matrix antigen and implications for virus assembly. Nature 378: 743-747. doi: 10.1038/378743a0
![]() |
1. | JI-HUAN HE, QIAN YANG, CHUN-HUI HE, HAI-BIN LI, EERDUN BUHE, PULL-IN STABILITY OF A FRACTAL MEMS SYSTEM AND ITS PULL-IN PLATEAU, 2022, 30, 0218-348X, 10.1142/S0218348X22501857 |