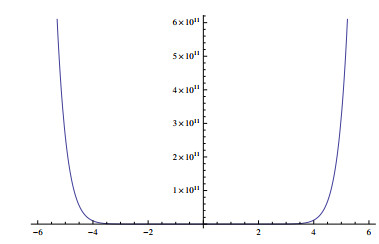
In this paper, relying on the Banach contraction mapping principle to discuss the existence of solution for a multi-order nonlinear fractional differential equations on the infinite interval [0,+∞). Moreover the stability of Ulam-Hyers-Rassias and Ulam-Hyers to the initial value problem are obtained. An example that can illustrate the conclusions of this paper have been given at the end.
Citation: Leping Xie, Jueliang Zhou, Haiyun Deng, Yubo He. Existence and stability of solution for multi-order nonlinear fractional differential equations[J]. AIMS Mathematics, 2022, 7(9): 16440-16448. doi: 10.3934/math.2022899
[1] | Irina Di Ruocco . A political concept for the Gragnano Valley of Mills (Valle dei Mulini). Urban redevelopment of cultural-industrial heritage. Urban Resilience and Sustainability, 2023, 1(4): 278-308. doi: 10.3934/urs.2023018 |
[2] | Aibin Yan, Dinghan Zheng . Restoration and integration of the Huang Family Garden within the contemporary urban fabric of Shanghai. Urban Resilience and Sustainability, 2024, 2(1): 27-44. doi: 10.3934/urs.2024003 |
[3] | Elham Zabetian Targhi, Niusha Fardnava, Saba Saghafi . Urban development criteria with a focus on resilience to pandemics: A case study of coronavirus (Covid-19). Urban Resilience and Sustainability, 2023, 1(1): 66-85. doi: 10.3934/urs.2023005 |
[4] | Jichao Wang, Xiaoning Sui, Jie Zhang, Wenjie Shi, Wayne L. Thompson . Relocating disaster-prone villages and improving villager well-being: Evidence from Beijing, China. Urban Resilience and Sustainability, 2024, 2(3): 236-255. doi: 10.3934/urs.2024012 |
[5] | João C. G. Lanzinha . Rehabilitation of existing building parks and its relationship with urban agglomerates–An urgent and demanding task for our common future. Urban Resilience and Sustainability, 2023, 1(2): 86-90. doi: 10.3934/urs.2023006 |
[6] | Adoyo Laji, Jeremiah N. Ayonga . Mainstreaming resilience to flood risk among households in informal settlements in Kisumu City, Kenya. Urban Resilience and Sustainability, 2024, 2(4): 326-347. doi: 10.3934/urs.2024017 |
[7] | Maria Helena Luengo-Duque . Erasing roots: The impact of urban development on historical memory and identity in San Juan. Urban Resilience and Sustainability, 2025, 3(1): 26-56. doi: 10.3934/urs.2025002 |
[8] | Erik Velasco . Circular economy in Singapore: waste management, food and agriculture, energy, and transportation. Urban Resilience and Sustainability, 2024, 2(2): 110-150. doi: 10.3934/urs.2024007 |
[9] | Cheikh Cisse . At the crossroads of datas: Using artificial intelligence for efficient and resilient planning in African cities. Urban Resilience and Sustainability, 2023, 1(4): 309-313. doi: 10.3934/urs.2023019 |
[10] | Belhaj Naoufel . Determinants of cities' financial governance for sustainable urban development in Morocco—Case of the regions of Casablanca-Settat and Rabat-Sale-Kenitra. Urban Resilience and Sustainability, 2023, 1(1): 1-19. doi: 10.3934/urs.2023001 |
In this paper, relying on the Banach contraction mapping principle to discuss the existence of solution for a multi-order nonlinear fractional differential equations on the infinite interval [0,+∞). Moreover the stability of Ulam-Hyers-Rassias and Ulam-Hyers to the initial value problem are obtained. An example that can illustrate the conclusions of this paper have been given at the end.
The special polynomials and numbers play an important role in many branches of mathematics such as combinatorics, computer science, statistics, etc. The generating functions of the special polynomials are used in the investigation of numerous properties satisfied by polynomials. A large number of indispensable special polynomials such as the Bernoulli, Euler and monic Hermite polynomials belong to the Appell class of sequences. These polynomials play crucial roles in many aspects, most importantly, they provide solutions to certain specified problems of physics, engineering and mathematics (see for more details [5,6,7,8,9,21,22,34,35]).
Throughout this paper, we use the following standard notations: N:={1,2,3,⋯},N0:=N∪{0}:={0,1,2,3,⋯},Z−:={−1,−2,−3,⋯},Z denotes the set of integers and R denotes the set of real numbers.
The generating function of the Euler polynomials Eq(v) [23], which arise in various classical and numerical analysis (and also of analytic number theory) is defined as:
2ew+1evw=∞∑q=0Eq(v)wqq!,(|w|<π). | (1.1) |
The corresponding Euler numbers (appear in the Taylor series expansions of the secant and the hyperbolic secant functions) Eq:=Eq(0) are given as:
2ew+1=∞∑q=0Eqwqq!,(|w|<π). | (1.2) |
Recently, Kim et al., [31] introduced the Changhee polynomials and numbers and established certain explicit formulae and identities for these polynomials. The Changhee polynomials Chq(v) are specified by the following generating function [31]:
22+w(1+w)v=∞∑q=0Chq(v)wqq!, | (1.3) |
which for v=0 gives the corresponding Changhee numbers Chq:=Chq(0). Thereafter, Lee et al., [32] introduced a new form of Changhee polynomials, called the Appell-type Changhee polynomials Ch∗q(v), and defined by the following generating equation [32]:
22+wevw=∞∑q=0Ch∗q(v)wqq!, | (1.4) |
and have the following explicit relation [32,P3,Theorem 1]:
Ch∗q(v)=q∑p=0(qp)Ch∗qvq−p, | (1.5) |
where Ch∗q:=Ch∗q(0) are the corresponding Appell-type Changhee numbers:
22+w=∞∑q=0Ch∗qwqq!. | (1.6) |
The above Appell-type Changhee numbers Ch∗q satisfy the following relation [32,P4,Theorem 4]:
2Ch∗q+qCh∗q−1=0,in the case whenq≥1, | (1.7) |
with initial condition Ch∗0=1.
Motivated by the work of Kim et al., [24,26,27,28,29,30,31] and Lee et al., [31,32], in this paper we introduce the generating function of the Appell-type Changhee-Euler polynomials (ATCEP) and derive its properties.
In this section, the Appell-type Changhee-Euler polynomials (ATCEP), denoted by ChE∗q(v) are introduced and certain properties of these polynomials are established.
Definition 1. The Appell-type Changhee-Euler polynomials ChE∗q(v) are defined by the following generating relation:
4(ew+1)(2+w)evw=∞∑q=0ChE∗q(v)wqq!. | (2.1) |
Utilizing Eqs (1.1) and (1.6) in the left hand side of generating function (2.1) and then using Cauchy-product rule in the left hand side of the obtained equation, we get
∞∑q=0q∑l=0(ql)El(v)Ch∗q−lwqq!=∞∑q=0ChE∗q(v)wqq!. |
Comparing the coefficients of identical powers of w in both sides of the foregoing equation, we get the following series expansion for Appell-type Changhee-Euler polynomials ChE∗q(v):
q∑l=0(ql)El(v)Ch∗q−l=ChE∗q(v). | (2.2) |
Motivated by the importance of the Euler numbers [36,38] in various branches of mathematics specially in number theory and combinatorics, we introduce the numbers related to the polynomial families introduced here. Taking v=0 in series definition (2.2) of the ATCEP ChE∗q(v) and using notation:
ChE∗q:=ChE∗q(0), | (2.3) |
in the left hand side, we get the following series form for Appell-type Changhee-Euler number (ATCEN) ChE∗q:
q∑l=0(ql)ElCh∗q−l=ChE∗q. | (2.4) |
Also, we find the following generating functions for ATCEN ChE∗q:
4(ew+1)(2+w)=∞∑q=0ChE∗qwqq!. | (2.5) |
Theorem 2.1. Let u,v∈C and q∈N0, then the following addition formulas hold:
ChE∗q(u+v)=q∑p=0(qp)Ep(u)Ch∗q−p(v). | (2.6) |
ChE∗q(u+v)=q∑p=0(qp)uq−pChE∗p(v). | (2.7) |
Proof. Replacing v by u+v in Eq (2.1), we get
(2ew+1)(22+w)e(v+u)w=∞∑q=0ChE∗q(v+u)wqq!, | (2.8) |
which on using Eqs (1.4) and (1.1) in the l.h.s. and then on comparing the coefficients of the identical powers of w in both sides of the obtained relation, we get assertion (2.6).
Equation (2.8) can be written as
∞∑q=0ChE∗q(v+u)wqq!=4(ew+1)(2+w)evweuw, | (2.9) |
which on using Eq (2.1) and expanding the second exponential of the right side gives
∞∑q=0ChE∗q(v+u)wqq!=∞∑q=0(uw)qq!∞∑p=0ChE∗p(v)wpp!. | (2.10) |
Using the Cauchy product rule on the right side of Eq (2.10) and on comparing the coefficients of w, we are lead to assertion (2.7).
Theorem 2.2. Let q∈N0 and v∈C, then the following identities hold true:
2Eq(v)=2Ch∗Eq(v)+qChE∗q−1(v). | (2.11) |
2Ch∗q(v)=Ch∗Eq(v)+q∑p=o(qp)ChE∗q−p(v). | (2.12) |
ChE∗q(v)=q∑p=0(qp)(−1)pp!Eq−p(v)2p. | (2.13) |
ChE∗q(v)=q∑p=0(qp)ChE∗q−p(v)p. | (2.14) |
ChE∗q(v)=q∑p=0(qp)Ch∗q−p(v2)Ep(v2). | (2.15) |
ddvChE∗q(v)=qChE∗q−1(v). | (2.16) |
dpdvpChE∗q(v)=q!(q−p)!ChE∗q−p(v). | (2.17) |
∫vaChE∗q−1(u)du=1q(ChE∗q(v)−ChE∗q(a)). | (2.18) |
ChE∗q=q∑p=0(qp)(−v)pChE∗q−p(v). | (2.19) |
2Ch∗q=q∑p=0(qp)ChE∗q−p(v)(−v)p(1+(1−1v)p). | (2.20) |
Proof. These identities are easily derivable with the help of generating Eqs (1.1), (1.4) and (2.1) by manipulating double series. Now, rewriting the generating Eq (2.1) as
4(ew+1)(2+w)=∞∑q=0ChE∗q(v)wqq!(e−vw), | (2.21) |
which on using generating relation (2.5) in the l.h.s. and expanding the exponential on the r.h.s. and then on equating the coefficients of identical powers of w in both sides, we are led to assertion (2.19).
From Eq (2.21), we have
42+w=∞∑q=0ChE∗q(v)wqq!(e−vw(ew+1)), | (2.22) |
which on using generating relation (1.6) in l.h.s. and expanding the exponentials on the r.h.s. and then on equating the coefficients of identical powers of w in both sides of the obtained result, yields assertion (2.20).
Many researchers have established the determinant form of certain special and q-special polynomials [4,12,16,18,19,20,33]. Costabile [10,11,13] has given several approaches to Bernoulli polynomials. An important approach based on a determinant definition was given in [10]. This approach is further extended to provide determinant definitions of the Appell and Sheffer polynomial sequences by Costabile and Longo in [12] and [14] respectively. Motivated by the recent work on the determinant approaches, here we give the determinant representation of ATCEP ChE∗q(v). We recall the determinant form of the Euler polynomials Eq(v):
The Euler polynomials Eq(v) of degree q=0,1,2,⋯ are defined by
E0(v)=1,Eq(v)=(−1)q|1vv2⋯vq−1vq11212⋯12120112(21)⋯12(q−11)12(q1)001⋯12(q−12)12(q2)...⋯.....⋯..000⋯112(qq−1)|,q=1,2,.... | (2.23) |
Definition 2. The Appell-type Changhee-Euler polynomials ChE∗q(v) of degree q are defined by
ChE∗0(v)=1,ChE∗q(v)=(−1)q|1Ch∗1(v)Ch∗2(v)⋯Ch∗q−1(v)Ch∗q(v)11212⋯12120112(21)⋯12(q−11)12(q1)001⋯12(q−12)12(q2)...⋯.....⋯..000⋯112(qq−1)|,q=1,2,.... | (2.24) |
where the Appell-type Changhee polynomials Ch∗q(v)(q=0,1,2,...) are given by Eq (1.4).
In the forthcoming section, a family of linear differential equations arising from the generating function of ATCEN ChE∗q is derived by following the approach presented in [26].
Differential equations, besides playing important role in pure mathematics, constitute fundamental part of mathematical description of physical processes. Thus, obtaining the solutions for differential equations is of paramount importance. Few types of differential equations allow explicit and straightforward analytical solutions. Many researchers obtained the differential equations for special polynomials and numbers utilizing their generating functions [17,25,30].
Theorem 3.1. For n∈N the following family of differential equations for Appell-type Changhee-Euler polynomials ChE∗q(v) holds true:
n∑j=1βj(n)ewG(j−1)(w)+(ew+1)G(n)(w)=(−1)nβ0(n)(2+w)(n+1), | (3.1) |
where G(n)(w):=(ddw)nG(w),
G=G(w)=4(ew+1)(2+w), | (3.2) |
β0(n)=4n!,β1(n)=1,βn(n)=n, | (3.3) |
and
βi(n+1)=1+n−i+1∑j1=0βi−1(n−j1),(2≤i≤n). | (3.4) |
Proof. Let
G=G(w)=4(ew+1)(2+w), |
which can be rewritten as:
(ew+1)G(w)=42+w. | (3.5) |
Taking the derivative of Eq (3.5) with respect to w, it follows that:
(ew+1)G(1)(w)+ewG(w)=(−1)4(2+w)2, | (3.6) |
Again, by taking the derivative with respect to w of Eq (3.6), we find
(ew+1)G(2)(w)+2ewG(1)(w)+ewG(w)=(−1)28(2+w)3, | (3.7) |
and consequently
(ew+1)G(3)(w)+3ewG(2)(w)+3ewG(1)(w)+ewG(w)=(−1)324(2+w)4. | (3.8) |
Continuing this process, we find
n∑j=1βj(n)ewG(j−1)(w)+(ew+1)G(n)(w)=(−1)nβ0(n)(2+w)(n+1). | (3.9) |
Further, taking the derivative with respect to w of Eq (3.9), we get
β1(n)ewG(w)+n∑j=2ew(βj(n)+βj−1(n))G(j−1)(w)+(βn(n)+1)ewG(n)(w)+(ew+1)G(n+1)(w)=(−1)n+1(n+1)β0(n)(2+w)−(n+2). | (3.10) |
Replacing n by n+1, Eq (3.9) may be rewritten as:
n+1∑j=1βj(n+1)ewG(j−1)(w)+(ew+1)G(n+1)(w)=(−1)n+1β0(n+1)(2+w)(n+2). | (3.11) |
Comparison of the coefficients of G(i)'s on both sides of Eqs (3.10) and (3.11), yields the following recursive formulas:
β0(n+1)=(n+1)β0(n), | (3.12) |
β1(n+1)=β1(n), | (3.13) |
βj(n+1)=βj(n)+βj−1(n),(2≤j≤n) | (3.14) |
and
βn+1(n+1)=βn(n)+1. | (3.15) |
From Eqs (3.6) and (3.9), we have
β0(1)=4,β1(1)=1. | (3.16) |
Again, from Eq (3.12), we note that
β0(n+1)=(n+1)β0(n)=(n+1)nβ0(n)=...=(n+1)n(n−1)...2.1β0(1), |
that is
β0(n+1)=4(n+1)!. | (3.17) |
In view of Eq (3.13), we have
β1(n+1)=β1(n)=β1(n−1)=...=β1(1)=1. | (3.18) |
Further, from Eq (3.15), it follows that:
βn+1(n+1)=βn(n)+1=βn−1(n−1)+2=...=β1(1)+n, |
that is
βn+1(n+1)=1+n. | (3.19) |
Taking j=2 in Eq (3.14), we find
β2(n+1)=β2(n)+β1(n)=β2(n−1)+β1(n−1)+β1(n) |
=...=n−2∑j=0β1(n−j)+β2(2), |
that is
β2(n+1)=1+n−1∑j=0β1(n−j). | (3.20) |
Similarly, for j=3 Eq (3.14) gives
β3(n+1)=1+n−2∑j=0β2(n−j), | (3.21) |
consequently for j=4, we have
β4(n+1)=1+n−3∑j=0β3(n−j). | (3.22) |
Continuing this process, we deduce that
βi(n+1)=1+n−i+1∑j1=0βi−1(n−j1),(2≤j≤n). | (3.23) |
Equation (3.9) together with Eqs (3.17), (3.18) and (3.23) completes the proof of Theorem 3.1.
Theorem 3.2. For i,n∈N,p∈N0 the following identity for the Appell-type Changhee-Euler numbers ChE∗q holds true:
n∑j=1i∑p=0(ip)βj(n)ChE∗p+j−1+i∑p=0(ip)ChE∗p+n+ChE∗i+n=(−1)n+i(n+1)i(β0(n)2n+i+1), | (3.24) |
where (n+1)i is the pochhammer symbol [37,P21] and βj(n),(0≤j≤n) defined in Eqs (3.3) and (3.4).
Proof. Making use of Eq (2.5) in the first term of the left hand side of Eq (3.1), we find
n∑j=1βj(n)ewG(j−1)(w)=n∑j=1βj(n)∞∑l=0wll!∞∑p=0ChE∗p+j−1wpp!, |
or equivalently
n∑j=1βj(n)ewG(j−1)(w)=∞∑i=0(n∑j=1i∑p=0(ip)βj(n)ChE∗p+j−1)wii!. | (3.25) |
Again, using Eq (2.5) in the second term of the left hand side of Eq (3.1), we find
(ew+1)G(n)(w)=(∞∑p=0wpp!+1)((ddw)n∞∑l=0ChE∗lwll!), |
that is
(ew+1)G(n)(w)=∞∑i=0(i∑p=0(ip)ChE∗p+n+ChE∗i+n)wii!. | (3.26) |
Finally, from the right hand side of Eq (3.1), we have
β0(n)(−1)n(2+w)−(n+1)=β0(n)(−1)n2−(n+1)(1+w/2)−(n+1), |
which on using the following identity [37,P34]:
(1−z)−a=∞∑n=0(a)nznn!, |
gives
β0(n)(−1)n(2+w)−(n+1)=∞∑i=0((−1)n+iβ0(n)(n+1)i2n+i+1)wii!. | (3.27) |
Use of Eqs (3.25)–(3.27) in Eq (3.1), yields assertion (3.24).
In the next section, the graphical representations of the ATCEP ChE∗q(v) and distribution of their zeros are discussed.
Over the years, there has been increasing interest in solving mathematical problems with the aid of computers. The software Mathematica is used to show the behaviour of these newly introduced polynomials using the graphs plotted for the special values of indices. The manual computation of the zeros is too complicated, therefore, we use Mathematica to investigate the zeros of the ATCEP ChE∗q(v). By using numerical investigations and computer experiments, we find the real and complex zeros for certain values of index q. The investigation in this direction will lead to a new approach employing numerical methods in the field of the hybrid special polynomials to appear in mathematics and physics, see for example [18,19].
With the help of Mathematica and using Eqs (1.5) and (1.7) in relation (2.2), Figures 1 and 2 are drawn for odd values of index q:
Similarly, for even values of index q, Figures 3 and 4 are drawn:
Next, a remarkably regular structure of the zeros of ATCEP ChE∗q(v) are explored with the help of Mathematica in Figures 5–8.
Further properties, applications and theoretical investigation of scattering of zeros of the ATCEP ChE∗q(v) introduced here are left to the authors and the interested researchers, for future study.
Exclusive role have been played by special polynomials in applied mathematics. It is not astonishing when new classes of special polynomials are established as the issues associated with special polynomials are too immense. In this paper, by incorporating the Appell-type Changhee polynomials Ch∗q(v) and the Euler polynomials Eq(v) in a natural way, the so-called Appell-type Changhee-Euler polynomials ChE∗q(v) are introduced. Then, we investigate certain properties and identities for these new polynomials such as series representation, determinant form and some novel identities. We also present non-homogeneous linear differential equations for the Appell-type Changhee-Euler numbers ChE∗q. Further we discuss the shape and zero distributions of these polynomials by observing their graphs drawn by Mathematica. The ATCEP ChE∗q(v) were found to be the member of well-known Appell family and therefore many important properties and identities for this new polynomials were easily established by employing those in the well-developed theory of Appell polynomials. A unifying tool for studying polynomial sequences, namely the representation of Appell polynomials in matrix form has been studied in [1] and extended in [3] to the hypercomplex case. After that, Aceto and Caçāo [2] extends this approach to find the matrix representation of the Sheffer polynomials. The matrix which represents the Sheffer polynomial coefficients can be factorized into two matrices, one associated to Appell polynomials and the other linked to the binomial type polynomial sequences. This approach can be further extended to find the matrix representations of mixed special polynomials related to the Appell polynomials.
Posing a problem
If we consider the Genocchi polynomials [15] in place of Euler polynomials in Eq (2.1), we get the following generating function for the Appell-type Changhee-Genocchi polynomials (ATCGP) ChG∗q(v):
4w(ew+1)(2+w)evw=∞∑q=0ChG∗q(v)wqq!. | (5.1) |
We are believed to be able to establish the corresponding results for the Appell-type Changhee-Genocchi polynomials ChG∗q(v) as those established in this paper. This posed problem is left to the interested researchers and authors for further investigation.
The authors are thankful to the reviewers for several useful comments and suggestions towards the improvement of this paper.
No conflict of interest was declared by the authors.
[1] |
W. Tan, W. Pan, M. Xu, A note on unsteady flows of a viscoelastic fluid with fractional Maxwell model between two parallel plates, Int. J. Nonlin. Mech., 38 (2003), 645–650. http://dx.doi.org/10.1016/S0020-7462(01)00121-4 doi: 10.1016/S0020-7462(01)00121-4
![]() |
[2] | A. Kilbas, H. Srivastava, J. Trujillo, Theory and applications of fractional differential equations, Boston: Elsevier, 2006. |
[3] | S. Ray, Fractional calculus with applications for nuclear reactor dynamics, Boca Raton: CRC Press, 2015. http://dx.doi.org/10.1201/b18684 |
[4] |
L. Liu, Q. Dong, G. Li, Exact solutions and Hyers-Ulam stability for fractional oscillation equations with pure delay, Appl. Math. Lett., 112 (2021), 106666. http://dx.doi.org/10.1016/j.aml.2020.106666 doi: 10.1016/j.aml.2020.106666
![]() |
[5] |
C. Chen, M. Bohner, B. Jia, Ulam-Hyers stability of Caputo fractional difference equations, Math. Method. Appl. Sci., 42 (2019), 7461–7470. http://dx.doi.org/10.1002/mma.5869 doi: 10.1002/mma.5869
![]() |
[6] |
S. Peng, J. Wang, Existence and Ulam-Hyers stability of ODEs involving two Caputo fractional derivatives, Electron. J. Qual. Theory Differ. Equ., 52 (2015), 1–16. http://dx.doi.org/10.14232/ejqtde.2015.1.52 doi: 10.14232/ejqtde.2015.1.52
![]() |
[7] |
Z. Akbar, W. Ali, S. Farina, Hyers-Ulam stability of nonlinear differential equations with fractional integrable impulses, Math. Method. Appl. Sci., 40 (2017), 5502–5514. http://dx.doi.org/10.1002/mma.4405 doi: 10.1002/mma.4405
![]() |
[8] |
Z. Yang, T. Xu, M. Qi, Ulam-Hyers stability for fractional differential equations in quaternionic analysis, Adv. Appl. Clifford Algebras, 26 (2016), 469–478. http://dx.doi.org/10.1007/s00006-015-0576-3 doi: 10.1007/s00006-015-0576-3
![]() |
[9] | M. Akkouchi, Hyers-Ulam-Rassias stability of nonlinear volterra integral equations via a fixed point approach, Acta Universitatis Apulensis, 26 (2011), 257–266. |
[10] |
J. Wang, Y. Zhang, Ulam-Hyers-Mittag-Leffler stability of fractional-order delay differential equations, Optimization, 63 (2014), 1181–1190. http://dx.doi.org/10.1080/02331934.2014.906597 doi: 10.1080/02331934.2014.906597
![]() |
[11] |
M. Abdoab, S. Panchala, H. Wahash, Ulam-Hyers-Mittag-Leffler stability for a psi-Hilfer problem with fractional order and infinite delay, Results in Applied Mathematics, 7 (2020), 100115. http://dx.doi.org/10.1016/j.rinam.2020.100115 doi: 10.1016/j.rinam.2020.100115
![]() |
[12] |
M. Abdo, T. Abdeljawad, K. Shah, S. Ali, On nonlinear coupled evolution system with nonlocal subsidiary conditions under fractal-fractional order derivative, Math. Method. Appl. Sci., 44 (2021), 6581–6600. http://dx.doi.org/10.1002/mma.7210 doi: 10.1002/mma.7210
![]() |
[13] |
S. Ali, M. Abdo, Qualitative analysis for multiterm Langevin systems with generalized Caputo fractional operators of different orders, Math. Probl. Eng., 2022 (2022), 1879152. http://dx.doi.org/10.1155/2022/1879152 doi: 10.1155/2022/1879152
![]() |
[14] |
M. Abdo, S. Panchal, H. Shafei Hussien, Fractional integro-differential equations with nonlocal conditions and ψ-Hilfer fractional derivative, Math. Model. Anal., 24 (2019), 564–584. http://dx.doi.org/10.3846/mma.2019.034 doi: 10.3846/mma.2019.034
![]() |
[15] |
H. Wahash, M. Abdo, S. Panchal, Fractional integrodifferential equations with nonlocal conditions and generalized Hilfer fractional derivative, Ufa Math. J., 11 (2019), 151–170. http://dx.doi.org/10.13108/2019-11-4-151 doi: 10.13108/2019-11-4-151
![]() |
[16] |
J. Sun, Y. Zhao, Multiplicity of positive solutions of a class of nonlinear fractional differential equations, Comput. Math. Appl., 49 (2005), 73–80. http://dx.doi.org/10.1016/j.camwa.2005.01.006 doi: 10.1016/j.camwa.2005.01.006
![]() |
[17] |
J. Zhou, S. Zhang, Y. He, Existence and stability of solution for nonlinear differential equations with ψ-Hilfer fractional derivative, Appl. Math. Lett., 121 (2021), 107457. http://dx.doi.org/10.1016/j.aml.2021.107457 doi: 10.1016/j.aml.2021.107457
![]() |
[18] |
J. Zhou, S. Zhang, Y. He, Existence and stability of solution for a nonlinear fractional differential equation, J. Math. Anal. Appl., 498 (2021), 124921. http://dx.doi.org/10.1016/j.jmaa.2020.124921 doi: 10.1016/j.jmaa.2020.124921
![]() |
[19] |
X. Su, S. Zhang, Unbounded solutions to a boundary value problem of fractional order on the half-line, Comput. Math. Appl., 61 (2011), 1079–1087. http://dx.doi.org/10.1016/j.camwa.2010.12.058 doi: 10.1016/j.camwa.2010.12.058
![]() |
[20] |
X. Su, Solutions to boundary value problem of fractional order on unbounded domains in a Banach space, Nonlinear Anal.-Theor., 74 (2011), 2844–2852. http://dx.doi.org/10.1016/j.na.2011.01.006 doi: 10.1016/j.na.2011.01.006
![]() |
[21] |
C. Kou, H. Zhou, Y. Yan, Existence of solutions of initial value problems for nonlinear fractional differential equations on the half-axis, Nonlinear Anal.-Theor., 74 (2011), 5975–5986. http://dx.doi.org/10.1016/j.na.2011.05.074 doi: 10.1016/j.na.2011.05.074
![]() |
[22] |
J. Diaz, B. Margolis, A fixed point theorem of the alternative for contractions on a generalized complete metric space, Bull. Amer. Math. Soc., 74 (1968), 305–309. http://dx.doi.org/10.1090/S0002-9904-1968-11933-0 doi: 10.1090/S0002-9904-1968-11933-0
![]() |
[23] |
L. C˘adariu, L. G˘avruţa, P. G˘avruţa, Weighted space method for the stability of some nonlinear equations, Appl. Anal. Discr. Math., 6 (2012), 126–139. http://dx.doi.org/10.2298/AADM120309007C doi: 10.2298/AADM120309007C
![]() |