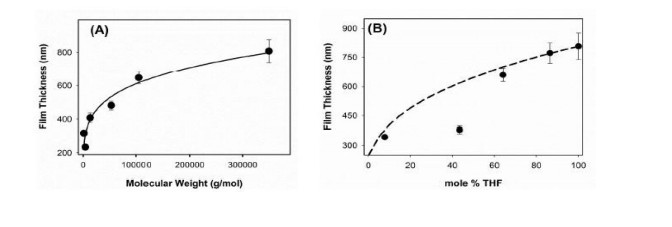
Citation: Alessandro Didonna, Federico Benetti. Post-translational modifications in neurodegeneration[J]. AIMS Biophysics, 2016, 3(1): 27-49. doi: 10.3934/biophy.2016.1.27
[1] | Stephan A. Brinckmann, Nishant Lakhera, Chris M. Laursen, Christopher Yakacki, Carl P. Frick . Characterization of poly(para-phenylene)-MWCNT solvent-cast composites. AIMS Materials Science, 2018, 5(2): 301-319. doi: 10.3934/matersci.2018.2.301 |
[2] | Thomas J. Lee, Andrew H. Morgenstern, Thomas A. Höft, Brittany B. Nelson-Cheeseman . Dispersion of particulate in solvent cast magnetic thermoplastic polyurethane elastomer composites. AIMS Materials Science, 2019, 6(3): 354-362. doi: 10.3934/matersci.2019.3.354 |
[3] | Hyeck Go, Eun-Mi Han, Moon Hee Kang, Yong Hyun Kim, Changhun Yun . The coated porous polyimide layers for optical scattering films. AIMS Materials Science, 2018, 5(6): 1102-1111. doi: 10.3934/matersci.2018.6.1102 |
[4] | Wiebke Langgemach, Andreas Baumann, Manuela Ehrhardt, Thomas Preußner, Edda Rädlein . The strength of uncoated and coated ultra-thin flexible glass under cyclic load. AIMS Materials Science, 2024, 11(2): 343-368. doi: 10.3934/matersci.2024019 |
[5] | Xuan Luc Le, Nguyen Dang Phu, Nguyen Xuan Duong . Enhancement of ferroelectricity in perovskite BaTiO3 epitaxial thin films by sulfurization. AIMS Materials Science, 2024, 11(4): 802-814. doi: 10.3934/matersci.2024039 |
[6] | Neerajkumar Wayzode, Vinod Suryawanshi . Mechanical properties of graphene nanoplatelets reinforced glass/epoxy composites manufactured using resin film infusion process. AIMS Materials Science, 2023, 10(4): 693-709. doi: 10.3934/matersci.2023038 |
[7] | Uichi Akiba, Jun-ichi Anzai . Cyclodextrin-containing layer-by-layer films and microcapsules: Synthesis and applications. AIMS Materials Science, 2017, 4(4): 832-846. doi: 10.3934/matersci.2017.4.832 |
[8] | Elena Díaz, Rafael Gutiérrez, Christopher Gaul, Gianaurelio Cuniberti, Francisco Domínguez-Adame . Coherent spin dynamics in a helical arrangement of molecular dipoles. AIMS Materials Science, 2017, 4(5): 1052-1061. doi: 10.3934/matersci.2017.5.1052 |
[9] | Daria Wehlage, Robin Böttjer, Timo Grothe, Andrea Ehrmann . Electrospinning water-soluble/insoluble polymer blends. AIMS Materials Science, 2018, 5(2): 190-200. doi: 10.3934/matersci.2018.2.190 |
[10] | Mahmoud A Rabah . Lead, zinc and copper fine powder with controlled size and shape. AIMS Materials Science, 2017, 4(6): 1358-1371. doi: 10.3934/matersci.2017.6.1358 |
In the present state of materials science, where there is a push for devices to be made smaller, it is important to understand a material’s physical and chemical properties from both the perspective of the surface and the bulk. It is well documented that a material can behave differently when comparing these two regions of the same substance [1,2,3,4,5], which is often attributed to confinement effects. Furthermore, an additional area becomes of importance when the surfaces of two different materials interact to form an interface. A defining feature of an interface is the surface morphology, which is a broad term and can be considered on many different scales. In modern thin film systems one must consider the nano/micrometer regions of surface morphology as well as the macro features. Changing and controlling surface morphology through the use of etching and lithography in hard materials such as silicon wafers has been well studied and understood for some time now [6,7,8]. However, polymers are playing a larger role in devices and new methods are needed to control surface morphology. The push for understanding and controlling surface morphologies historically arises from the field of adhesion [9,10], but certain patterning in materials has been shown to be promising for use in photonic devices [11,12,13,14,15,16,17,18,19,20], stretchable microelectronics [21,22], mechanically switchable wetting [23], and microfluidics [24,25]. Methods currently used for producing surface patterns in soft materials include thin film dewetting [26,27,28], electrohydrodynamic patterning [29,30], breath figures [31,32], thermal-gradient induced patterning [33,34], self-assembly and microphase separation [28,35,36] electron irradiation [37] but most commonly used is template based patterning [38,39,40]. Template based patterning can be used to create various different types of geometric structures such as hexagons, stripes, or rectangles, however the most common use of templating is the production of wrinkles (also known as striations or corrugations) on a surface [41,42,43,44]. To induce wrinkling on a surface, a layer of elastomer, typically polydimethylsiloxane (PDMS), is prestrained mechanically or thermally. A rigid skin layer is then transferred onto the elastomer and the strain is released, which causes buckling of the skin layer and produces wrinkles, creases, or folds.
Herein we report a simple spin casting method that can be used to fabricate polystyrene thin films that have different surface morphologies. Modification of the surface morphology of polymer films has long been studied with the goal of controlling the structure [45,46,47,48,49,50,51,52]. Wrinkles in the PS films form spontaneously without the use of PDMS, away from the center of the sample. The surface morphology, as determined by the wavelength and amplitude of the periodic structures, can be controlled by the spin-speed, solvent composition, and the polymer molecular weight but the solution concentration has minimal effect on these parameters. We find that a high evaporation rate is required to obtain a periodic surface morphology while a low evaporation rate leads to a random surface structure. This is consistent with previous studies showing the role of solvent evaporation of polymer solutions under non-spinning conditions [53].
The parameters required to understand spin-cast films have been studied for a long time [54,55] and are reasonably well understood. Several solvent properties influence the final film characteristics, including vapor pressure, density, and molecular weight. Standard spin-coating theory [54,55] predicts smooth films but with the observation of wrinkle formation during spin-coating [50,51,56] new theories were developed, primarily to understand how to prepare uniform films rather than create nanostructured surfaces [57,58,59,60,61]. Two different mechanism were proposed to explain the wrinkle formation. De Gennes [58] suggested that as evaporation occurred a thin layer of a glassy polymer formed on the surface that buckled upon final drying. In contrast, Birnie [60] developed a model based on the Marangoni effect, which arises from differential evaporation that induces a stress field that lead to disruptions that control the final surface structure. Birnie [60] determined that the surface morphology was controlled by the relative effects of evaporation rate and surface tension. Experimental work supports the Marangoni model [57,59,61,62,63,64] although there are still unanswered questions [61]. Our results support the Birnie model [60] which adds sinusoidal perturbations to standard thickness models.
PS has been shown to show nonuniform and periodic surface morphologies when cast from single component good solvents [39,47,49]. We find that the wrinkle amplitude and wavelengths scale with the dry film thickness but are independent of the concentration of polymer when spin-cast from pure THF. In contrast, a solvent system composed of THF mixed with dimethylformamide (DMF), two solvents that have about the same molecular weight, density, and surface tension but drastically different vapor pressure, periodic structures are only found for THF-rich compositions. The role of solvent composition has not been reported previously.
Glass (borosilicate) slides were cut into 3.75 × 1.75 cm pieces (2 mm thick). These were then cleaned first by sonication in 95% ethanol (EtOH, Pharmaco-Aaper) for 15 min, then by sonication in DI water for 15 min, and finally dried with N2. Polystyrene (PS, Polymer Source Inc.) solutions were made in solvent (tetrahydrofuran (THF, Sigma Aldrich, HPLC Grade) or THF/dimethylformamide (DMF, Sigma Aldrich, HPLC Grade) mixtures. Each solution was sonicated for 4 h or until the entire polymer was dissolved. The PS films were made by spin-casting using fresh solutions. A 500 μL aliquot of the PS solution was deposited in the center of the glass substrate. Spin-casting was done at room temperature under a N2 blanket (to remove humidity effects) with an acceleration of 1080 rad·s-2 for all solutions but different final rotation rates and spin times were used. To achieve different PS film thickness either the spin speed or the PS concentration was varied. After spinning, the films were placed in a 60 ℃ oven for 1 min to dry.
The film thickness of the PS was determined using a Filmetrics F40 microscope via reflection spectroscopy. The reflection spectrum was recorded with a tungsten-halogen light source over the range of 400-900 nm. The resulting interference pattern was then fit using the known refractive index of the polymer to give the average film thickness. After the film thickness was determined, the surface morphology of the PS films was analyzed using a Filmetrics Profilm 3D Optical Profiler. The images of the surface were obtained using either the vertical scanning interferometry (VSI) or phase shifting interferometry (PSI) modes. The evaporation rates of PS/DMF/THF solutions were measured by placing a 0.5 mL aliquot of solution on a glass substrate on a balance (23 ℃, 20% humidity). The mass was recorded as a function of time until the mass leveled off.
The spin coating method for fabricating thin films is often used because it is a simple method to create uniform films of a desired thickness. However, the actual dynamics of spin casting are complicated, as the final product and quality of the film depend on many factors. As Bornside et al. [55] have demonstrated, the final thickness of a dry film, ho, can be estimated through the following equation,
$ {h_o} = \chi _{pol}^o{\left[ {{\eta _o}\left( {\frac{{c{D_g}}}{{{\nu _g}^{1/2}\rho }}} \right)\left( {\frac{{{p_{sol}}{M_{sol}}}}{{RT}}} \right)\left( {1 - \chi _{pol}^o - \chi _{sol}^\infty } \right)} \right]^{1/3}}\frac{1}{{{\omega ^{1/2}}}} $ | (1) |
where ηo is the initial viscosity of the solution, χopol is the mass fraction of polymer in the initial solution, ${\chi ^\infty }_{sol}$ is the mass fraction of the solvent in the gas phase at equilibrium, νg is the kinematic velocity of the overlying gas, c is a constant that depends upon the Schmidt constant, Dg is the diffusivity of the overlying gas, ρ is the density of the solution, psol is the vapor pressure of the solvent, Msol is the molecular weight of the solvent, R and T represent the ideal gas constant and absolute temperature, and ω is the angular speed. Eq 1 predicts that several experimental parameters, including solution concentration, polymer molecular weight, solvent volatility, and spin speed, can be used to change the film thickness. The success of Eq 1 in predicting the thickness dependence with respect to rotation rate and polymer concentration has been well documented [54,55] and we confirm these dependencies for polystyrene, as shown in Figures S1 and S2 in the Supplementary Information.
While Eq 1 does not show an explicit dependence on the molecular weight, the initial viscosity will depend on both the concentration and the molecular weight. The Mark-Houwink relationship is $\eta_{o} \propto M_{p o l}^{\alpha}$, where the exponent α is polymer dependent and has an accepted value of α = 0.70 for PS in THF [62]. Isolating all of the variables in Eq 1 except the molecular weight implies that ho should scale as ηo1/3 = (Mαpol)1/3, i.e. a power law of the form ho = aMβpol, where a and β = α/3 are fitting parameters. Figure 1a shows the measured PS film thickness as a function of PS molecular weight, while all other experimental parameters are kept constant. As shown in Figure 1b, the best fit for the power law, a = 53 ± 20 nm and β = 0.21 ± 0.03 = α/3, giving α = 0.63 ± 0.09, in reasonable agreement with the accepted value.
To examine the solvent influence on the film thickness we chose a THF/DMF mixed solvent system. As shown in Table 1, the key solvent parameters relevant to spin-coating as expressed in Eq 1 are similar for THF and DMF, within a few per cent, except solvent vapor pressure and boiling point, which are related quantities. Other than the vapor pressure, the biggest difference in the relevant solvent parameters is the viscosity, but this difference will be negated in solution, where the polymer concentration will dominate the initial viscosity. Assuming that the vapor pressure follows Raoult’s Law, and combining other parameters in Eq 1 into a single variable, the thickness can be written as $h_{o} = a^{\prime}\left(\mathrm{X}_{D M F} p_{D M F}^{o}+\mathrm{X}_{T H F} p_{T H F}^{o}\right)^{\frac{1}{3}}$, where X is mole fraction and po is the vapor pressure of the pure solvent and a’ is an arbitrary parameter. Figure 1b shows the experimental data and the fit to the simplified equation with a’ = 310 ± 35 nm.
Property | THF | DMF |
Molecular weight (g/mol) | 72.1 | 73.1 |
Density (g/cm3) | 0.888 | 0.945 |
Molar volume (cm3/mol) | 81.08 | 77.43 |
Boiling point (℃) | 66 | 153 |
Vapor pressure (kPa, 21 ℃) | 17.7 | 0.507 |
Viscosity (mPa·s, 25 ℃) | 0.55 | 0.82 |
Surface tension (mN/m, 20 ℃) | 28 | 35 |
Equation 1 gives a good description of the average thickness as shown by the data and fits in Figure 1. However, closer examination of the films shows that the surface is not smooth so we pursued this further. Figure 2 shows the surface structures of the outer edges of PS films measured by optical profilometry as a function of solvent composition and molecular weight. When the solvent is primarily THF, there is a well-defined periodic surface morphology. When the solvent is DMF rich, the surface is rough but with no defined wave forms. A mixed solvent that is composed of 70/30 (v/v) THF/DMF overlays both of these surface structures where there is a roughness superimposed on a broad and shallow wave. This implies that to attain periodicity the solvent must be relatively volatile at the casting temperature or have a low boiling point.
The evaporation rate of PS solutions was measured under non-spinning conditions, as shown in Figure 3. The data clearly show that the THF and DMF evaporate independently, which is expected based on the large difference in boiling points and vapor pressures. There is no evidence of azeotrope formation. Further, the evaporation rate is the same for all compositions for each component: for THF this is 0.0421 ± 0.0002 g/s and for DMF this is (1.40 ± 0.01) × 10-3 g/s. In the 70%THF/30%DMF solvent mixture there is a deviation from the DMF evaporation rate at long times not observed in the other data. We have no explanation for this deviation, although it may be related to a nonideality deviation from Raoult’s Law. For all cases the mass change levels off at ~0.02 g, which corresponds to the remaining polymer from the 4% (w/v) solution. In the solutions that are THF rich the evaporation is more than 50% complete within ~10 s, which means that the initial structure of the surface is fixed quickly. In contrast, in DMF-rich solutions the evaporation time is much longer so the initial surface structure has more time to reconfigure. The result is that the initial periodicity is lost while the surface still has some roughness. While these evaporation results are for non-spinning conditions, the trends are expected to be the same under rotation. This is consistent with the work of Schaefer et al. [53], who showed that the length scale of polymer thin film morphology depends on the evaporation rate of the solvent. They showed that fast evaporation rates lead to kinetic controlled structures while long evaporation rates lead to thermodynamic controlled structures.
Figure 4a shows the surface roughness as a function of solvent composition for films composed of PS with molecular weight of 350 kg/mol. As the fraction of THF in the solvent increases the surface roughness increases. This is true independent of the rotation rate. This implies that the nonuniform surface structure forms early in the coating process and only slowly relaxes into more uniform films as long as there is solvent still present. This is consistent with the static evaporation data. To further test the role of the solvent volatility, the spinning time was varied. Assuming that surface structures are randomly formed in the absence of rotation, then a short spin time will capture the structure promoted by the initial evaporation, which should be dominated by the more volatile component of the mixed solvent。
Under solvent conditions with a high concentration of THF (>70% v/v) the film surface structure is periodic, as shown in Figure 2. Line scans were extracted from the surface profiles and fit to a function of the form
$ h(x) = h_{o}+a_{o} \sin \left(\frac{2 \pi x}{\lambda_{o}}\right) $ | (2) |
where h(x) is the height along the position x of the line scan, ho is the average height as described in Eq 1, ao is the amplitude of the wave, and λo is the wavelength. Examples of fits are shown in the Supplementary Information (Figures S3-S13). The amplitudes and wavelengths found from fitting to Eq 2 for changing the rotation rate for films created using a 4% w/v PS (molecular weight = 350 kg/mol) solution of a solvent composed of 90% THF/10% DMF (v/v) are shown in Figure 4b. All films appear dry after even the shortest spin time, but the presence or absence of solvent trapped in the films was not determined. As the residence time on the spinner increases both the wavelength and amplitude of the wrinkle structure decrease. Again, this supports the conclusion that the surface structures are formed early in the drying process.
Figure 5 shows the molecular weight dependence of the wavelengths and amplitudes of wrinkles at a constant film thickness of 310 ± 10 nm. At low molecular weights, Mn < 13 kg/mol, the periodicity is not well defined with amplitudes less than 25 nm and wavelengths in the range of 50-75 μm. Increasing Mn to the range of 53 kg/mol ≤ Mn ≤ 105 kg/mol, the wrinkles become more defined, the heights increase to 50-120 nm and the wavelengths increase to 90-115 μm. Finally, when Mn > 105 kg/mol the wavelengths decrease markedly, leveling out to about 75 μm. For Mn > 105 kg/mol the amplitudes also decrease but only by a small amount compared to the maximum and are approximately constant, ~75 nm, for Mn > 150 kg/mol.
The changes in the wrinkle amplitude and wavelengths as a function of rotation rate are shown in Figure 6. As the spin rate increases, both the wavelength and amplitude decrease. Both of these parameters scale as $\omega^{-1 / 2}$, as shown by the solid line in Figure 6, which could imply that the surface morphology scales as the film thickness.
To test this conclusion, the film thickness was changed by varying the concentration of the polymer at constant rotation rate, as shown in Figure 7. Under these conditions, the wavelength and amplitude remains constant. Since the film thickness increases with increasing solution concentration (as shown in Figure S2), the data in Figure 7 demonstrate that the amplitude and wavelength of the periodic structures arise independently from the final film thickness. This then requires that the changes in observed as a function of spinning speed shown in Figure 7 are not associated with the final film thickness.
While the average thickness of PS spin-cast films is well described by the Bornside et al. [55], model as embodied in Eq 1, this model does not account for the periodic surface morphology observed. To account for the wrinkles the model proposed by Birnie [60] is used. In the Birnie model the Marangoni effect, which is based on differential evaporation of the solvent at different spatial locations, is invoked to impose a perturbation on the initial polymer solution. The model assumes that the height of the fluid solution follows a simple sinusoidal variation:
$ H\mathit{(}x\mathit{)} = H_{o}+A \sin (k x) $ | (3) |
where H(x) is the fluid height at different locations x, Ho is the initial fluid height, A is the amplitude of the fluid modulation, and k is the wavenumber of the periodic perturbation. In relation to the wavelengths we have measured using Eq 2, k = 2π/λo for the final film thickness. In order for an evaporation modulation to occur, the model [60] assumes a surface tension modulation in phase with the height modulation
$ \sigma\mathit{(}x\mathit{)} = \sigma_{o}+B \sin (k x) $ | (4) |
where σ(x) is the surface tension at location x, σo is the initial solution surface tension, B is the modulation amplitude of the surface tension, and the other symbols are as previously defined. The surface tension is assumed to change linearly with the fractional polymer concentration, which leads to the relation
$ C_{p}(x) = C_{p}^{o}+C \sin (k x) $ | (5) |
where Cp(x) is the fractional polymer concentration at location x, $C_p^o$ is the initial fractional polymer concentration, and C is the fractional modulation of the polymer concentration. Within the Birnie [60] model the observables from our study can be found as
$ k_{max }^{2} = \frac{3 B}{4 A H_{o} \sigma_{o}} = \frac{4 \pi^{2}}{\lambda_{o}^{2}} $ | (6) |
$ a_{o} = C_{p}^{o} H_{o}\left(\frac{c}{c_{p}^{o}}+\frac{A}{H_{o}}\right) $ | (7) |
The values of A, B, and C may be time dependent so cannot be evaluated as independent parameters. The terms $C_p^o$, σo, and Ho are experimentally controllable by varying the polymer concentration, solvent, and solution volume, respectively.
Qualitatively, Eqs 6 and 7 can account for the observed wavelengths and amplitudes with some caveats. The data shown in Figures 6-8 indicate that wrinkle amplitudes and wavelengths depend on the polymer molecular weight and the rotation rate but not the initial polymer concentration. The molecular weight dependence is embodied in the initial surface tension, which could account for the observed behavior. How the amplitude parameters A, B, and C vary with molecular weight is not known, so also could contribute to the observed dependencies. The rotation rate dependence of the surface morphology is likely related to evaporation rate changes, which will influence the composition parameter C. The lack of change of the observed amplitudes with initial polymer concentration is puzzling, within the context of this theory, since Eq 7 has an explicit dependence on this parameter. If Eq 7 is rewritten as ao = HoC + $C_p^o$A, it may be that A is sufficiently small that the initial concentration does not contribute significantly.
Observation of all of the data in Figures 5-7 show that λo and ao show parallel dependencies on initial conditions. Combining Eqs 6 and 7 gives the following,
$ \lambda_{o}^{2} = \frac{16 \pi^{2} A \sigma_{o}}{3 B C} a_{o}-\frac{16 \pi^{2} A^{2} C_{p}^{o} \sigma_{o}}{3 B C} $ | (8) |
which is linear for some initial conditions. Using data for a constant initial concentration and constant molecular weight, Figure 8 shows the relationship between λo2 and ao is approximately linear. Using the linear fit parameters gives the intercept/slope ratio = -A$C_p^o$ = -11 nm. The initial polymer concentration fraction, $C_p^o$ = 0.022, allows an estimate of A = 500 nm. The initial fluid height can be found from the substrate surface area, 3.75 cm × 1.75 cm, and the solution volume, 500 μL, gives Ho = 760 μm. This implies that the initial amplitude variation in the polymer solution, = A/Ho, is on the order of 7 × 10-4, which is reasonable for a small perturbation. Since the wrinkle amplitude is approximately independent of polymer concentration, ~90 nm from Figure 7, then using Eq 7 give the value for C = 1.2 × 10-4, which is the fractional variation of the concentration. Using Eq 6 and λo ~ 80 μm (from Figure 7) gives B = 88 mN/m, i.e. the surface tension modulation is 3 times larger than the solvent surface tension.
Within the context of Birnie’s model [60], this implies that a periodic surface structure is attained quickly, achieved by the initial stress at the onset of evaporation, and requires a fast evaporation rate to lock in the structure. Thus, the compositional modulation of the final dry film is primarily driven by the lateral surface tension variations of the solution as it spins. In the THF/DMF solvent system, the surface tensions of the pure solvents are similar so the changes in surface tension must be driven by concentration changes of the polymer as the solution evaporates. Further, the Birnie model [60] predicts that the time dependence of the surface tension and compositional sinusoidal amplitudes are proportional to the solvent evaporation rate. This leads to the conclusion that the amplitude of the surface wrinkle will be larger for shorter spin times. This is consistent with our data, where both the amplitude and the wavelength are larger for shorter spin times.
The surface morphology of spun cast polystyrene thin films has been studied using optical profilometry. The average film thicknesses can be accounted for by a standard model, including the role of molecular weight, rotation rate, solution concentration, and solvent composition. However, none of the PS films are smooth. Rather, for solvent compositions with fast evaporation times the surface spontaneously forms linear wrinkles that exhibit periodicity that can be described with sine waves having wavelengths on the order of tens of microns and amplitudes on the order of tens of nanometers. It was found the surface morphology changed as a function of the molecular weight of polystyrene and the rotation rate used to cast the film but was not influenced by the concentration of the polymer solution. In contrast, when the solvent evaporation rate is slow, the surfaces have reduced roughness and no periodicity, which is the classical expectation for spin coated thin films. The formation of the surface morphology was attributed to the Marangoni effect, which relies on a differential evaporation across the surface of the drying fluid. At fast evaporation rates found when the THF concentration is high, the anisotropic forces derived from the Marangoni effect prevail, leading to the kinetic structure, which are sinusoidal wrinkles. When the DMF concentration is high the evaporation rate is too low so only the isotropic rough structure is found. Quantitatively, the development of the periodic surface structure was treated as a perturbation from the average thickness, as reported in the literature, and the periodicity parameters were shown to be consistent with this interpretation. However, how this initial structure develops is still unresolved. The spatial variation in surface tension is expected to drive the final morphology, but why the perturbation should be sinusoidal is unknown. However, the underlying fundamental parameters still need to be established. The wavelengths and amplitudes of wavelike structures showed good reproducibility, which means that the perturbation parameters must be defined functions of the experimental conditions but none of these relationships are known. Both further experimental and theoretical investigations are needed to establish these relationships. The reproducibility of the periodicity is potentially useful for preparing defined surfaces. The surface morphology can be used to control the structure and spectroscopic properties of surface bound chromophores, an application that will be explored in subsequent studies.
CT thanks the Opening Foundation of Key Laboratory for Processing of Sugar Resources of Guangxi Higher Education Institutes (2016TZYKF08) and the Scholarship Fund of Guangxi University of Science and Technology of Strengthening Foundation Program of Guangxi Higher Education Institutes in 2016 for support. This material is based upon work supported by the U.S. Department of Homeland Security, Science and Technology Directorate, Office of University Programs, under Grant Award 2013-ST-061-ED0001. The views and conclusions contained in this document are those of the authors and should not be interpreted as necessarily representing the official policies, either expressed or implied, of the U.S. Department of Homeland Security.
All authors declare no conflicts of interest in this paper.
[1] |
Consortium IHGS (2004) Finishing the euchromatic sequence of the human genome. Nature 431: 931–945. doi: 10.1038/nature03001
![]() |
[2] |
Yura K, Shionyu M, Hagino K, et al. (2006) Alternative splicing in human transcriptome: functional and structural influence on proteins. Gene 380: 63–71. doi: 10.1016/j.gene.2006.05.015
![]() |
[3] |
Jensen ON (2004) Modification-specific proteomics: characterization of post-translational modifications by mass spectrometry. Curr Opin Chem Biol 8: 33–41. doi: 10.1016/j.cbpa.2003.12.009
![]() |
[4] | Khoury GA, Baliban RC, Floudas CA (2011) Proteome-wide post-translational modification statistics: frequency analysis and curation of the swiss-prot database. Sci Rep 1: 90. |
[5] | Nestler EJ, Greengard P (1999) Protein phosphorylation is of fundamental importance in biological Regulation, In: Siegel G, Agranoff B, Albers R, et al., editors. Basic Neurochemistry: Molecular, Cellular and Medical Aspects. Philadelphia: Lippincott-Raven. |
[6] |
Hunter T (1995) Protein kinases and phosphatases: the yin and yang of protein phosphorylation and signaling. Cell 80: 225–236. doi: 10.1016/0092-8674(95)90405-0
![]() |
[7] |
Williams DR (2006) Tauopathies: classification and clinical update on neurodegenerative diseases associated with microtubule-associated protein tau. Intern Med J 36: 652–660. doi: 10.1111/j.1445-5994.2006.01153.x
![]() |
[8] |
Goedert M, Spillantini MG, Jakes R, et al. (1989) Multiple isoforms of human microtubule-associated protein tau: sequences and localization in neurofibrillary tangles of Alzheimer's disease. Neuron 3: 519–526. doi: 10.1016/0896-6273(89)90210-9
![]() |
[9] | Mandelkow EM, Mandelkow E (2012) Biochemistry and cell biology of tau protein in neurofibrillary degeneration. Cold Spring Harb Perspect Med 2: a006247. |
[10] |
Hanger DP, Seereeram A, Noble W (2009) Mediators of tau phosphorylation in the pathogenesis of Alzheimer's disease. Expert Rev Neurother 9: 1647–1666. doi: 10.1586/ern.09.104
![]() |
[11] |
Gong CX, Iqbal K (2008) Hyperphosphorylation of microtubule-associated protein tau: a promising therapeutic target for Alzheimer disease. Curr Med Chem 15: 2321–2328. doi: 10.2174/092986708785909111
![]() |
[12] |
Tanimukai H, Grundke-Iqbal I, Iqbal K (2005) Up-regulation of inhibitors of protein phosphatase-2A in Alzheimer's disease. Am J Pathol 166: 1761–1771. doi: 10.1016/S0002-9440(10)62486-8
![]() |
[13] |
Wang J, Tung YC, Wang Y, et al. (2001) Hyperphosphorylation and accumulation of neurofilament proteins in Alzheimer disease brain and in okadaic acid-treated SY5Y cells. FEBS Lett 507: 81–87. doi: 10.1016/S0014-5793(01)02944-1
![]() |
[14] |
Ulloa L, Montejo de Garcini E, Gomez-Ramos P, et al. (1994) Microtubule-associated protein MAP1B showing a fetal phosphorylation pattern is present in sites of neurofibrillary degeneration in brains of Alzheimer's disease patients. Brain Res Mol Brain Res 26: 113–122. doi: 10.1016/0169-328X(94)90081-7
![]() |
[15] | Lindwall G, Cole RD (1984) Phosphorylation affects the ability of tau protein to promote microtubule assembly. J Biol Chem 259: 5301–5305. |
[16] |
Jeganathan S, Hascher A, Chinnathambi S, et al. (2008) Proline-directed pseudo-phosphorylation at AT8 and PHF1 epitopes induces a compaction of the paperclip folding of Tau and generates a pathological (MC-1) conformation. J Biol Chem 283: 32066–32076. doi: 10.1074/jbc.M805300200
![]() |
[17] | Tenreiro S, Eckermann K, Outeiro TF (2014) Protein phosphorylation in neurodegeneration: friend or foe? Front Mol Neurosci 7: 42. |
[18] |
Sato-Harada R, Okabe S, Umeyama T, et al. (1996) Microtubule-associated proteins regulate microtubule function as the track for intracellular membrane organelle transports. Cell Struct Funct 21: 283–295. doi: 10.1247/csf.21.283
![]() |
[19] |
Cowan CM, Bossing T, Page A, et al. (2010) Soluble hyper-phosphorylated tau causes microtubule breakdown and functionally compromises normal tau in vivo. Acta Neuropathol 120: 593–604. doi: 10.1007/s00401-010-0716-8
![]() |
[20] |
Hoover BR, Reed MN, Su J, et al. (2010) Tau mislocalization to dendritic spines mediates synaptic dysfunction independently of neurodegeneration. Neuron 68: 1067–1081. doi: 10.1016/j.neuron.2010.11.030
![]() |
[21] |
Bancher C, Lassmann H, Budka H, et al. (1989) An antigenic profile of Lewy bodies: immunocytochemical indication for protein phosphorylation and ubiquitination. J Neuropathol Exp Neurol 48: 81–93. doi: 10.1097/00005072-198901000-00007
![]() |
[22] | Thomas B, Beal MF (2007) Parkinson's disease. Hum Mol Genet 16 Spec No. 2: R183–194. |
[23] |
Bendor JT, Logan TP, Edwards RH (2013) The function of alpha-synuclein. Neuron 79: 1044–1066. doi: 10.1016/j.neuron.2013.09.004
![]() |
[24] |
Waxman EA, Giasson BI (2011) Characterization of kinases involved in the phosphorylation of aggregated alpha-synuclein. J Neurosci Res 89: 231–247. doi: 10.1002/jnr.22537
![]() |
[25] |
Anderson JP, Walker DE, Goldstein JM, et al. (2006) Phosphorylation of Ser-129 is the dominant pathological modification of alpha-synuclein in familial and sporadic Lewy body disease. J Biol Chem 281: 29739–29752. doi: 10.1074/jbc.M600933200
![]() |
[26] |
Paleologou KE, Oueslati A, Shakked G, et al. (2010) Phosphorylation at S87 is enhanced in synucleinopathies, inhibits alpha-synuclein oligomerization, and influences synuclein-membrane interactions. J Neurosci 30: 3184–3198. doi: 10.1523/JNEUROSCI.5922-09.2010
![]() |
[27] |
Cavallarin N, Vicario M, Negro A (2010) The role of phosphorylation in synucleinopathies: focus on Parkinson's disease. CNS Neurol Disord Drug Targets 9: 471–481. doi: 10.2174/187152710791556140
![]() |
[28] |
Gorbatyuk OS, Li S, Sullivan LF, et al. (2008) The phosphorylation state of Ser-129 in human alpha-synuclein determines neurodegeneration in a rat model of Parkinson disease. Proc Natl Acad Sci U S A 105: 763–768. doi: 10.1073/pnas.0711053105
![]() |
[29] |
Kragh CL, Lund LB, Febbraro F, et al. (2009) Alpha-synuclein aggregation and Ser-129 phosphorylation-dependent cell death in oligodendroglial cells. J Biol Chem 284: 10211–10222. doi: 10.1074/jbc.M809671200
![]() |
[30] | Azeredo da Silveira S, Schneider BL, Cifuentes-Diaz C, et al. (2009) Phosphorylation does not prompt, nor prevent, the formation of alpha-synuclein toxic species in a rat model of Parkinson's disease. Hum Mol Genet 18: 872–887. |
[31] |
Schreurs S, Gerard M, Derua R, et al. (2014) In vitro phosphorylation does not influence the aggregation kinetics of WT alpha-synuclein in contrast to its phosphorylation mutants. Int J Mol Sci 15: 1040–1067. doi: 10.3390/ijms15011040
![]() |
[32] |
Xu Y, Deng Y, Qing H (2015) The phosphorylation of alpha-synuclein: development and implication for the mechanism and therapy of the Parkinson's disease. J Neurochem 135: 4–18. doi: 10.1111/jnc.13234
![]() |
[33] |
Waxman EA, Giasson BI (2008) Specificity and regulation of casein kinase-mediated phosphorylation of alpha-synuclein. J Neuropathol Exp Neurol 67: 402–416. doi: 10.1097/NEN.0b013e3186fc995
![]() |
[34] |
Goncalves S, Outeiro TF (2013) Assessing the subcellular dynamics of alpha-synuclein using photoactivation microscopy. Mol Neurobiol 47: 1081–1092. doi: 10.1007/s12035-013-8406-x
![]() |
[35] |
Kontopoulos E, Parvin JD, Feany MB (2006) Alpha-synuclein acts in the nucleus to inhibit histone acetylation and promote neurotoxicity. Hum Mol Genet 15: 3012–3023. doi: 10.1093/hmg/ddl243
![]() |
[36] |
Scott D, Roy S (2012) alpha-Synuclein inhibits intersynaptic vesicle mobility and maintains recycling-pool homeostasis. J Neurosci 32: 10129–10135. doi: 10.1523/JNEUROSCI.0535-12.2012
![]() |
[37] |
McFarland MA, Ellis CE, Markey SP, et al. (2008) Proteomics analysis identifies phosphorylation-dependent alpha-synuclein protein interactions. Mol Cell Proteomics 7: 2123–2137. doi: 10.1074/mcp.M800116-MCP200
![]() |
[38] |
Didonna A, Opal P (2015) The promise and perils of HDAC inhibitors in neurodegeneration. Ann Clin Transl Neurol 2: 79–101. doi: 10.1002/acn3.147
![]() |
[39] |
Lilja T, Heldring N, Hermanson O (2013) Like a rolling histone: epigenetic regulation of neural stem cells and brain development by factors controlling histone acetylation and methylation. BBA-Gen Subjects 1830: 2354–2360. doi: 10.1016/j.bbagen.2012.08.011
![]() |
[40] |
Cheung P, Allis CD, Sassone-Corsi P (2000) Signaling to chromatin through histone modifications. Cell 103: 263–271. doi: 10.1016/S0092-8674(00)00118-5
![]() |
[41] |
Campuzano V, Montermini L, Molto MD, et al. (1996) Friedreich's ataxia: autosomal recessive disease caused by an intronic GAA triplet repeat expansion. Science 271: 1423–1427. doi: 10.1126/science.271.5254.1423
![]() |
[42] |
Kumari D, Biacsi RE, Usdin K (2011) Repeat expansion affects both transcription initiation and elongation in friedreich ataxia cells. J Biol Chem 286: 4209–4215. doi: 10.1074/jbc.M110.194035
![]() |
[43] |
Kim E, Napierala M, Dent SY (2011) Hyperexpansion of GAA repeats affects post-initiation steps of FXN transcription in Friedreich's ataxia. Nucleic Acids Res 39: 8366–8377. doi: 10.1093/nar/gkr542
![]() |
[44] | Pietrobono R, Tabolacci E, Zalfa F, et al. (2005) Molecular dissection of the events leading to inactivation of the FMR1 gene. Hum Mol Genet 14: 267–277. |
[45] |
Opal P, Zoghbi HY (2002) The role of chaperones in polyglutamine disease. Trends Mol Med 8: 232–236. doi: 10.1016/S1471-4914(02)02310-9
![]() |
[46] |
Sugars KL, Rubinsztein DC (2003) Transcriptional abnormalities in Huntington disease. Trends Genet 19: 233–238. doi: 10.1016/S0168-9525(03)00074-X
![]() |
[47] |
Li F, Macfarlan T, Pittman RN, et al. (2002) Ataxin-3 is a histone-binding protein with two independent transcriptional corepressor activities. J Biol Chem 277: 45004–45012. doi: 10.1074/jbc.M205259200
![]() |
[48] |
Cvetanovic M, Rooney RJ, Garcia JJ, et al. (2007) The role of LANP and ataxin 1 in E4F-mediated transcriptional repression. EMBO Rep 8: 671–677. doi: 10.1038/sj.embor.7400983
![]() |
[49] |
Venkatraman A, Hu YS, Didonna A, et al. (2014) The histone deacetylase HDAC3 is essential for Purkinje cell function, potentially complicating the use of HDAC inhibitors in SCA1. Hum Mol Genet 23: 3733–3745. doi: 10.1093/hmg/ddu081
![]() |
[50] |
Hammond JW, Cai D, Verhey KJ (2008) Tubulin modifications and their cellular functions. Curr Opin Cell Biol 20: 71–76. doi: 10.1016/j.ceb.2007.11.010
![]() |
[51] |
Hubbert C, Guardiola A, Shao R, et al. (2002) HDAC6 is a microtubule-associated deacetylase. Nature 417: 455–458. doi: 10.1038/417455a
![]() |
[52] |
North BJ, Marshall BL, Borra MT, et al. (2003) The human Sir2 ortholog, SIRT2, is an NAD+-dependent tubulin deacetylase. Mol Cell 11: 437–444. doi: 10.1016/S1097-2765(03)00038-8
![]() |
[53] |
Chen S, Owens GC, Makarenkova H, et al. (2010) HDAC6 regulates mitochondrial transport in hippocampal neurons. PLoS One 5: e10848. doi: 10.1371/journal.pone.0010848
![]() |
[54] |
Hempen B, Brion JP (1996) Reduction of acetylated alpha-tubulin immunoreactivity in neurofibrillary tangle-bearing neurons in Alzheimer's disease. J Neuropathol Exp Neurol 55: 964–972. doi: 10.1097/00005072-199609000-00003
![]() |
[55] |
Dompierre JP, Godin JD, Charrin BC, et al. (2007) Histone deacetylase 6 inhibition compensates for the transport deficit in Huntington's disease by increasing tubulin acetylation. J Neurosci 27: 3571–3583. doi: 10.1523/JNEUROSCI.0037-07.2007
![]() |
[56] |
d'Ydewalle C, Krishnan J, Chiheb DM, et al. (2011) HDAC6 inhibitors reverse axonal loss in a mouse model of mutant HSPB1-induced Charcot-Marie-Tooth disease. Nat Med 17: 968–974. doi: 10.1038/nm.2396
![]() |
[57] |
Min SW, Cho SH, Zhou Y, et al. (2010) Acetylation of tau inhibits its degradation and contributes to tauopathy. Neuron 67: 953–966. doi: 10.1016/j.neuron.2010.08.044
![]() |
[58] |
Min SW, Chen X, Tracy TE, et al. (2015) Critical role of acetylation in tau-mediated neurodegeneration and cognitive deficits. Nat Med 21: 1154–1162. doi: 10.1038/nm.3951
![]() |
[59] |
Cohen TJ, Guo JL, Hurtado DE, et al. (2011) The acetylation of tau inhibits its function and promotes pathological tau aggregation. Nat Commun 2: 252. doi: 10.1038/ncomms1255
![]() |
[60] |
Christiansen MN, Chik J, Lee L, et al. (2014) Cell surface protein glycosylation in cancer. Proteomics 14: 525–546. doi: 10.1002/pmic.201300387
![]() |
[61] |
Spiro RG (2002) Protein glycosylation: nature, distribution, enzymatic formation, and disease implications of glycopeptide bonds. Glycobiology 12: 43R–56R. doi: 10.1093/glycob/12.4.43R
![]() |
[62] | Aebi M (2013) N-linked protein glycosylation in the ER. BBA-Mol Cell Res 1833: 2430–2437. |
[63] |
Prusiner SB (1998) Prions. Proc Natl Acad Sci U S A 95: 13363–13383. doi: 10.1073/pnas.95.23.13363
![]() |
[64] |
Taraboulos A, Rogers M, Borchelt DR, et al. (1990) Acquisition of protease resistance by prion proteins in scrapie-infected cells does not require asparagine-linked glycosylation. Proc Natl Acad Sci U S A 87: 8262–8266. doi: 10.1073/pnas.87.21.8262
![]() |
[65] |
Lawson VA, Collins SJ, Masters CL, et al. (2005) Prion protein glycosylation. J Neurochem 93: 793–801. doi: 10.1111/j.1471-4159.2005.03104.x
![]() |
[66] |
Somerville RA (1999) Host and transmissible spongiform encephalopathy agent strain control glycosylation of PrP. J Gen Virol 80: 1865–1872. doi: 10.1099/0022-1317-80-7-1865
![]() |
[67] |
Safar J, Wille H, Itri V, et al. (1998) Eight prion strains have PrP(Sc) molecules with different conformations. Nat Med 4: 1157–1165. doi: 10.1038/2654
![]() |
[68] |
Lawson VA, Priola SA, Meade-White K, et al. (2004) Flexible N-terminal region of prion protein influences conformation of protease-resistant prion protein isoforms associated with cross-species scrapie infection in vivo and in vitro. J Biol Chem 279: 13689–13695. doi: 10.1074/jbc.M303697200
![]() |
[69] |
Steen PVd, Rudd PM, Dwek RA, et al. (1998) Concepts and principles of O-linked glycosylation. Crit Rev Biochem Mol Biol 33: 151–208. doi: 10.1080/10409239891204198
![]() |
[70] |
Comer FI, Hart GW (2001) Reciprocity between O-GlcNAc and O-phosphate on the carboxyl terminal domain of RNA polymerase II. Biochemistry 40: 7845–7852. doi: 10.1021/bi0027480
![]() |
[71] |
Lefebvre T, Guinez C, Dehennaut V, et al. (2005) Does O-GlcNAc play a role in neurodegenerative diseases? Expert Rev Proteomics 2: 265–275. doi: 10.1586/14789450.2.2.265
![]() |
[72] | Robertson LA, Moya KL, Breen KC (2004) The potential role of tau protein O-glycosylation in Alzheimer's disease. J Alzheimers Dis 6: 489–495. |
[73] |
Mayor S, Riezman H (2004) Sorting GPI-anchored proteins. Nat Rev Mol Cell Biol 5: 110–120. doi: 10.1038/nrm1309
![]() |
[74] | Taylor DR, Hooper NM (2010) GPI-anchored proteins in health and disease. In: Vidal CJ, editor. Post-translational Modifications in Health and Disease. New York: Springer, 39–55. |
[75] |
Campana V, Sarnataro D, Zurzolo C (2005) The highways and byways of prion protein trafficking. Trends Cell Biol 15: 102–111. doi: 10.1016/j.tcb.2004.12.002
![]() |
[76] |
Agostini F, Dotti CG, Perez-Canamas A, et al. (2013) Prion protein accumulation in lipid rafts of mouse aging brain. PLoS One 8: e74244. doi: 10.1371/journal.pone.0074244
![]() |
[77] |
Taraboulos A, Scott M, Semenov A, et al. (1995) Cholesterol depletion and modification of COOH-terminal targeting sequence of the prion protein inhibit formation of the scrapie isoform. J Cell Biol 129: 121–132. doi: 10.1083/jcb.129.1.121
![]() |
[78] |
Baron GS, Wehrly K, Dorward DW, et al. (2002) Conversion of raft associated prion protein to the protease-resistant state requires insertion of PrP-res (PrP(Sc)) into contiguous membranes. EMBO J 21: 1031–1040. doi: 10.1093/emboj/21.5.1031
![]() |
[79] |
Chesebro B, Trifilo M, Race R, et al. (2005) Anchorless prion protein results in infectious amyloid disease without clinical scrapie. Science 308: 1435–1439. doi: 10.1126/science.1110837
![]() |
[80] |
McNally KL, Ward AE, Priola SA (2009) Cells expressing anchorless prion protein are resistant to scrapie infection. J Virol 83: 4469–4475. doi: 10.1128/JVI.02412-08
![]() |
[81] |
Munday AD, López JA (2007) Posttranslational protein palmitoylation promoting platelet purpose. Arterioscler Thromb Vasc Biol 27: 1496–1499. doi: 10.1161/ATVBAHA.106.136226
![]() |
[82] | Resh MD (1999) Fatty acylation of proteins: new insights into membrane targeting of myristoylated and palmitoylated proteins. BBA-Mol Cell Res 1451: 1–16. |
[83] |
Salaun C, Greaves J, Chamberlain LH (2010) The intracellular dynamic of protein palmitoylation. J Cell Biol 191: 1229–1238. doi: 10.1083/jcb.201008160
![]() |
[84] |
Zeidman R, Jackson CS, Magee AI (2009) Protein acyl thioesterases (Review). Mol Membr Biol 26: 32–41. doi: 10.1080/09687680802629329
![]() |
[85] |
Greaves J, Chamberlain LH (2007) Palmitoylation-dependent protein sorting. J Cell Biol 176: 249–254. doi: 10.1083/jcb.200610151
![]() |
[86] |
Yanai A, Huang K, Kang R, et al. (2006) Palmitoylation of huntingtin by HIP14 is essential for its trafficking and function. Nat Neurosci 9: 824–831. doi: 10.1038/nn1702
![]() |
[87] |
Bhattacharyya R, Barren C, Kovacs DM (2013) Palmitoylation of amyloid precursor protein regulates amyloidogenic processing in lipid rafts. J Neurosci 33: 11169–11183. doi: 10.1523/JNEUROSCI.4704-12.2013
![]() |
[88] |
Meckler X, Roseman J, Das P, et al. (2010) Reduced Alzheimer's disease ss-amyloid deposition in transgenic mice expressing S-palmitoylation-deficient APH1aL and nicastrin. J Neurosci 30: 16160–16169. doi: 10.1523/JNEUROSCI.4436-10.2010
![]() |
[89] |
Farazi TA, Waksman G, Gordon JI (2001) The biology and enzymology of protein N-myristoylation. J Biol Chem 276: 39501–39504. doi: 10.1074/jbc.R100042200
![]() |
[90] |
Martin DD, Ahpin CY, Heit RJ, et al. (2012) Tandem reporter assay for myristoylated proteins post-translationally (TRAMPP) identifies novel substrates for post-translational myristoylation: PKCepsilon, a case study. FASEB J 26: 13–28. doi: 10.1096/fj.11-182360
![]() |
[91] |
Martin DD, Heit RJ, Yap MC, et al. (2014) Identification of a post-translationally myristoylated autophagy-inducing domain released by caspase cleavage of huntingtin. Hum Mol Genet 23: 3166–3179. doi: 10.1093/hmg/ddu027
![]() |
[92] |
Martin DD, Ladha S, Ehrnhoefer DE, et al. (2015) Autophagy in Huntington disease and huntingtin in autophagy. Trends Neurosci 38: 26–35. doi: 10.1016/j.tins.2014.09.003
![]() |
[93] |
Ciechanover A (2005) Intracellular protein degradation: from a vague idea thru the lysosome and the ubiquitin–proteasome system and onto human diseases and drug targeting. Cell Death Differ 12: 1178–1190. doi: 10.1038/sj.cdd.4401692
![]() |
[94] |
Mukhopadhyay D, Riezman H (2007) Proteasome-independent functions of ubiquitin in endocytosis and signaling. Science 315: 201–205. doi: 10.1126/science.1127085
![]() |
[95] |
Schnell JD, Hicke L (2003) Non-traditional functions of ubiquitin and ubiquitin-binding proteins. J Biol Chem 278: 35857–35860. doi: 10.1074/jbc.R300018200
![]() |
[96] |
Zeng L-R, Vega-Sánchez ME, Zhu T, et al. (2006) Ubiquitination-mediated protein degradation and modification: an emerging theme in plant-microbe interactions. Cell Res 16: 413–426. doi: 10.1038/sj.cr.7310053
![]() |
[97] |
Marx J (2002) Ubiquitin Lives Up to Its Name. Science 297: 1792–1794 doi: 10.1126/science.297.5588.1792
![]() |
[98] | Schipper-Krom S, Juenemann K, Reits EA (2012) The ubiquitin-proteasome system in Huntington’s disease: are proteasomes impaired, initiators of disease, or coming to the rescue? Biochem Res Int 2012: 1–12. |
[99] |
Mitra S, Finkbeiner S (2008) The ubiquitin-proteasome pathway in Huntington's disease. Sci World J 8: 421–433. doi: 10.1100/tsw.2008.60
![]() |
[100] |
Kalchman MA, Graham RK, Xia G, et al. (1996) Huntingtin is ubiquitinated and interacts with a specific ubiquitin-conjugating enzyme. J Biol Chem 271: 19385–19394. doi: 10.1074/jbc.271.32.19385
![]() |
[101] |
Venkatraman P, Wetzel R, Tanaka M, et al. (2004) Eukaryotic proteasomes cannot digest polyglutamine sequences and release them during degradation of polyglutamine-containing proteins. Mol Cell 14: 95–104. doi: 10.1016/S1097-2765(04)00151-0
![]() |
[102] |
Suhr ST, Senut M-C, Whitelegge JP, et al. (2001) Identities of sequestered proteins in aggregates from cells with induced polyglutamine expression. J Cell Biol 153: 283–294. doi: 10.1083/jcb.153.2.283
![]() |
[103] |
Seo H, Sonntag K-C, Kim W, et al. (2007) Proteasome activator enhances survival of Huntington’s disease neuronal model cells. PloS one 2: e238. doi: 10.1371/journal.pone.0000238
![]() |
[104] |
Jia H, Kast RJ, Steffan JS, et al. (2012) Selective histone deacetylase (HDAC) inhibition imparts beneficial effects in Huntington's disease mice: implications for the ubiquitin–proteasomal and autophagy systems. Hum Mol Genet 21: 5280–5293. doi: 10.1093/hmg/dds379
![]() |
[105] |
Perry G, Friedman R, Shaw G, et al. (1987) Ubiquitin is detected in neurofibrillary tangles and senile plaque neurites of Alzheimer disease brains. Proc Natl Acad Sci U S A 84: 3033–3036. doi: 10.1073/pnas.84.9.3033
![]() |
[106] |
Salon ML, Pasquini L, Moreno MB, et al. (2003) Relationship between β-amyloid degradation and the 26S proteasome in neural cells. Exp Neurol 180: 131–143. doi: 10.1016/S0014-4886(02)00060-2
![]() |
[107] |
Almeida CG, Takahashi RH, Gouras GK (2006) β-Amyloid accumulation impairs multivesicular body sorting by inhibiting the ubiquitin-proteasome system. J Neurosci 26: 4277–4288. doi: 10.1523/JNEUROSCI.5078-05.2006
![]() |
[108] |
Zhao X, Yang J (2010) Amyloid-β peptide is a substrate of the human 20S proteasome. ACS Chem Neurosci 1: 655–660. doi: 10.1021/cn100067e
![]() |
[109] |
Van Leeuwen FW, de Kleijn DP, van den Hurk HH, et al. (1998) Frameshift mutants of β amyloid precursor protein and ubiquitin-B in Alzheimer's and Down patients. Science 279: 242–247. doi: 10.1126/science.279.5348.242
![]() |
[110] |
Chadwick L, Gentle L, Strachan J, et al. (2012) Review: Unchained maladie–a reassessment of the role of Ubb+ 1‐capped polyubiquitin chains in Alzheimer's disease. Neuropathol App Neurobiol 38: 118–131. doi: 10.1111/j.1365-2990.2011.01236.x
![]() |
[111] |
Lam YA, Pickart CM, Alban A, et al. (2000) Inhibition of the ubiquitin-proteasome system in Alzheimer's disease. Proc Natl Acad Sci U S A 97: 9902–9906. doi: 10.1073/pnas.170173897
![]() |
[112] |
Wilkinson K, Henley J (2010) Mechanisms, regulation and consequences of protein SUMOylation. Biochem J 428: 133–145. doi: 10.1042/BJ20100158
![]() |
[113] |
Riley BE, Zoghbi HY, Orr HT (2005) SUMOylation of the polyglutamine repeat protein, ataxin-1, is dependent on a functional nuclear localization signal. J Biol Chem 280: 21942–21948. doi: 10.1074/jbc.M501677200
![]() |
[114] |
Steffan JS, Agrawal N, Pallos J, et al. (2004) SUMO modification of Huntingtin and Huntington's disease pathology. Science 304: 100–104. doi: 10.1126/science.1092194
![]() |
[115] |
Poukka H, Karvonen U, Jänne OA, et al. (2000) Covalent modification of the androgen receptor by small ubiquitin-like modifier 1 (SUMO-1). Proc Natl Acad Sci U S A 97: 14145–14150. doi: 10.1073/pnas.97.26.14145
![]() |
[116] |
Chua JP, Reddy SL, Yu Z, et al. (2015) Disrupting SUMOylation enhances transcriptional function and ameliorates polyglutamine androgen receptor–mediated disease. J Clin Invest 125: 831–845. doi: 10.1172/JCI73214
![]() |
[117] |
Terashima T, Kawai H, Fujitani M, et al. (2002) SUMO-1 co-localized with mutant atrophin-1 with expanded polyglutamines accelerates intranuclear aggregation and cell death. Neuroreport 13: 2359–2364. doi: 10.1097/00001756-200212030-00038
![]() |
[118] | Geiger T, Clarke S (1987) Deamidation, isomerization, and racemization at asparaginyl and aspartyl residues in peptides. Succinimide-linked reactions that contribute to protein degradation. J Biol Chem 262: 785–794. |
[119] |
Yang H, Zubarev RA (2010) Mass spectrometric analysis of asparagine deamidation and aspartate isomerization in polypeptides. Electrophoresis 31: 1764–1772. doi: 10.1002/elps.201000027
![]() |
[120] |
Robinson AB, McKerrow JH, Cary P (1970) Controlled deamidation of peptides and proteins: an experimental hazard and a possible biological timer. Proc Natl Acad Sci U S A 66: 753–757. doi: 10.1073/pnas.66.3.753
![]() |
[121] | Watanabe A, Takio K, Ihara Y (1999) Deamidation and isoaspartate formation in smeared tau in paired helical filaments. Unusual properties of the microtubule-binding domain of tau. J Biol Chem 274: 7368–7378. |
[122] |
Dan A, Takahashi M, Masuda-Suzukake M, et al. (2013) Extensive deamidation at asparagine residue 279 accounts for weak immunoreactivity of tau with RD4 antibody in Alzheimer's disease brain. Acta Neuropathol Commun 1: 54. doi: 10.1186/2051-5960-1-54
![]() |
[123] |
Shimizu T, Watanabe A, Ogawara M, et al. (2000) Isoaspartate formation and neurodegeneration in Alzheimer's disease. Arch Biochem Biophys 381: 225–234. doi: 10.1006/abbi.2000.1955
![]() |
[124] | Yamamoto A, Takagi H, Kitamura D, et al. (1998) Deficiency in protein L-isoaspartyl methyltransferase results in a fatal progressive epilepsy. J Neurosci 18: 2063–2074. |
[125] |
O'Brien JT, Thomas A (2015) Vascular dementia. Lancet 386: 1698–1706. doi: 10.1016/S0140-6736(15)00463-8
![]() |
[126] |
Adav SS, Qian J, Ang YL, et al. (2014) iTRAQ quantitative clinical proteomics revealed role of Na(+)K(+)-ATPase and its correlation with deamidation in vascular dementia. J Proteome Res 13: 4635–4646. doi: 10.1021/pr500754j
![]() |
[127] |
Moss CX, Matthews SP, Lamont DJ, et al. (2005) Asparagine deamidation perturbs antigen presentation on class II major histocompatibility complex molecules. J Biol Chem 280: 18498–18503. doi: 10.1074/jbc.M501241200
![]() |
[128] |
Manoury B, Mazzeo D, Fugger L, et al. (2002) Destructive processing by asparagine endopeptidase limits presentation of a dominant T cell epitope in MBP. Nat Immunol 3: 169–174. doi: 10.1038/ni754
![]() |
[129] | Kim JK, Mastronardi FG, Wood DD, et al. (2003) Multiple sclerosis: an important role for post-translational modifications of myelin basic protein in pathogenesis. Mol Cell Proteomics 2: 453–462. |
[130] | Sayre LM, Moreira PI, Smith MA, et al. (2005) Metal ions and oxidative protein modification in neurological disease. Ann Ist Super Sanita 41: 143–164. |
[131] |
Stadtman ER (1992) Protein oxidation and aging. Science 257: 1220–1224. doi: 10.1126/science.1355616
![]() |
[132] | Cai Z, Yan L-J (2013) Protein oxidative modifications: beneficial roles in disease and health. J Biochem Pharmacol Res 1: 15–26. |
[133] |
Sayre LM, Perry G, Smith MA (1999) Redox metals and neurodegenerative disease. Curr Opin Chem Biol 3: 220–225. doi: 10.1016/S1367-5931(99)80035-0
![]() |
[134] | Sayre LM, Perry G, Atwood CS, et al. (2000) The role of metals in neurodegenerative diseases. Cell Mol Biol (Noisy-le-grand) 46: 731–741. |
[135] |
Uttara B, Singh AV, Zamboni P, et al. (2009) Oxidative stress and neurodegenerative diseases: a review of upstream and downstream antioxidant therapeutic options. Curr Neuropharmacol 7: 65–74. doi: 10.2174/157015909787602823
![]() |
[136] |
Gasperini L, Meneghetti E, Pastore B, et al. (2015) Prion protein and copper cooperatively protect neurons by modulating NMDA receptor through S-nitrosylation. Antioxid Redox Signal 22: 772–784. doi: 10.1089/ars.2014.6032
![]() |
[137] | Stadtman ER (2001) Protein oxidation in aging and age‐related diseases. Ann N Y Acad Sci 928: 22–38. |
[138] | Bizzozero OA (2009) Protein carbonylation in neurodegenerative and demyelinating CNS diseases. In: Lajtha A, Banik N, Ray S, editors. Handbook of Neurochemistry and Molecular Neurobiology. New York: Springer, 543–462. |
[139] |
Wong CM, Cheema AK, Zhang L, et al. (2008) Protein carbonylation as a novel mechanism in redox signaling. Circ Res 102: 310–318. doi: 10.1161/CIRCRESAHA.107.159814
![]() |
[140] |
Wong C-M, Bansal G, Marcocci L, et al. (2012) Proposed role of primary protein carbonylation in cell signaling. Redox Rep 17: 90–94. doi: 10.1179/1351000212Y.0000000007
![]() |
[141] |
Serviddio G, Di Venosa N, Federici A, et al. (2005) Brief hypoxia before normoxic reperfusion (postconditioning) protects the heart against ischemia-reperfusion injury by preventing mitochondria peroxyde production and glutathione depletion. FASEB J 19: 354–361. doi: 10.1096/fj.04-2338com
![]() |
[142] |
Lee JR, Kim JK, Lee SJ, et al. (2009) Role of protein tyrosine nitration in neurodegenerative diseases and atherosclerosis. Arch Pharm Res 32: 1109–1118. doi: 10.1007/s12272-009-1802-0
![]() |
[143] |
Reynolds MR, Reyes JF, Fu Y, et al. (2006) Tau nitration occurs at tyrosine 29 in the fibrillar lesions of Alzheimer's disease and other tauopathies. J Neurosci 26: 10636–10645. doi: 10.1523/JNEUROSCI.2143-06.2006
![]() |
[144] |
Reynolds MR, Berry RW, Binder LI (2005) Site-specific nitration differentially influences tau assembly in vitro. Biochemistry 44: 13997–14009. doi: 10.1021/bi051028w
![]() |
[145] |
Takahashi T, Yamashita H, Nakamura T, et al. (2002) Tyrosine 125 of alpha-synuclein plays a critical role for dimerization following nitrative stress. Brain Res 938: 73–80. doi: 10.1016/S0006-8993(02)02498-8
![]() |
[146] |
Hodara R, Norris EH, Giasson BI, et al. (2004) Functional consequences of alpha-synuclein tyrosine nitration: diminished binding to lipid vesicles and increased fibril formation. J Biol Chem 279: 47746–47753. doi: 10.1074/jbc.M408906200
![]() |
[147] |
Giasson BI, Duda JE, Murray IV, et al. (2000) Oxidative damage linked to neurodegeneration by selective alpha-synuclein nitration in synucleinopathy lesions. Science 290: 985–989. doi: 10.1126/science.290.5493.985
![]() |
[148] | Benhar M, Forrester MT, Stamler JS (2009) Protein denitrosylation: enzymatic mechanisms and cellular functions. Nat Rev Mol Cell Biol 10: 721–732. |
[149] |
Nakamura T, Tu S, Akhtar MW, et al. (2013) Aberrant protein s-nitrosylation in neurodegenerative diseases. Neuron 78: 596–614. doi: 10.1016/j.neuron.2013.05.005
![]() |
[150] |
Zhao Q-F, Yu J-T, Tan L (2015) S-Nitrosylation in Alzheimer's disease. Mol Neurobiol 51: 268–280. doi: 10.1007/s12035-014-8672-2
![]() |
[151] | Chung K, Dawson TM, Dawson VL (2005) Nitric oxide, S-nitrosylation and neurodegeneration. Cell Mol Biol (Noisy-le-Grand, France) 51: 247–254. |
[152] |
Maiti NR, Surewicz WK (2001) The role of disulfide bridge in the folding and stability of the recombinant human prion protein. J Biol Chem 276: 2427–2431. doi: 10.1074/jbc.M007862200
![]() |
[153] | Ning L, Guo J, Jin N, et al. (2014) The role of Cys179–Cys214 disulfide bond in the stability and folding of prion protein: insights from molecular dynamics simulations. J Mol Model 20: 1–8. |
[154] |
Benetti F, Biarnés X, Attanasio F, et al. (2014) Structural determinants in prion protein folding and stability. J Mol Biol 426: 3796–3810. doi: 10.1016/j.jmb.2014.09.017
![]() |
[155] |
Benetti F, Legname G (2015) New insights into structural determinants of prion protein folding and stability. Prion 9: 119–124. doi: 10.1080/19336896.2015.1022023
![]() |
[156] |
Shimura H, Hattori N, Kubo S-i, et al. (2000) Familial Parkinson disease gene product, parkin, is a ubiquitin-protein ligase. Nat Genet 25: 302–305. doi: 10.1038/77060
![]() |
[157] |
Andreu CI, Woehlbier U, Torres M, et al. (2012) Protein disulfide isomerases in neurodegeneration: from disease mechanisms to biomedical applications. FEBS Lett 586: 2826–2834. doi: 10.1016/j.febslet.2012.07.023
![]() |
[158] |
Smirnova E, Griparic L, Shurland D-L, et al. (2001) Dynamin-related protein Drp1 is required for mitochondrial division in mammalian cells. Mol Bio Cell 12: 2245–2256. doi: 10.1091/mbc.12.8.2245
![]() |
[159] |
Ohshima T, Ward JM, Huh C-G, et al. (1996) Targeted disruption of the cyclin-dependent kinase 5 gene results in abnormal corticogenesis, neuronal pathology and perinatal death. Proc Natl Acad Sci U S A 93: 11173–11178. doi: 10.1073/pnas.93.20.11173
![]() |
[160] |
Rhee SG, Kang SW, Jeong W, et al. (2005) Intracellular messenger function of hydrogen peroxide and its regulation by peroxiredoxins. Curr Opin Cell Biol 17: 183–189. doi: 10.1016/j.ceb.2005.02.004
![]() |
[161] |
Kim Y-S, Joh TH (2012) Matrix metalloproteinases, new insights into the understanding of neurodegenerative disorders. Biomol Ther 20: 133–143. doi: 10.4062/biomolther.2012.20.2.133
![]() |
[162] |
Chandrasekharan N, Simmons DL (2004) The cyclooxygenases. Genome Biol 5: 241. doi: 10.1186/gb-2004-5-9-241
![]() |
[163] |
Tristan C, Shahani N, Sedlak TW, et al. (2011) The diverse functions of GAPDH: views from different subcellular compartments. Cell Signal 23: 317–323. doi: 10.1016/j.cellsig.2010.08.003
![]() |
[164] | Ariga H, Takahashi-Niki K, Kato I, et al. (2013) Neuroprotective function of DJ-1 in Parkinson’s disease. Oxid Med Cell Longev 2013: 1–9. |
[165] |
Stroissnigg H, Trančíková A, Descovich L, et al. (2007) S-nitrosylation of microtubule-associated protein 1B mediates nitric-oxide-induced axon retraction. Nat Cell Biol 9: 1035–1045. doi: 10.1038/ncb1625
![]() |
[166] |
Tsang AH, Lee Y-I, Ko HS, et al. (2009) S-nitrosylation of XIAP compromises neuronal survival in Parkinson's disease. Proc Natl Acad Sci U S A 106: 4900–4905. doi: 10.1073/pnas.0810595106
![]() |
[167] |
Numajiri N, Takasawa K, Nishiya T, et al. (2011) On–off system for PI3-kinase–Akt signaling through S-nitrosylation of phosphatase with sequence homology to tensin (PTEN). Proc Natl Acad Sci U S A 108: 10349–10354. doi: 10.1073/pnas.1103503108
![]() |
[168] | Zito K, Scheuss V (2009) NMDA receptor function and physiological modulation. In: Squire, LR, editor. The New Encyclopedia of Neuroscience. Oxford: Academic Press, 1157–1164. |
[169] | Prabakaran S, Lippens G, Steen H, et al. (2012) Post‐translational modification: nature's escape from genetic imprisonment and the basis for dynamic information encoding. Wiley Interdiscip Rev Syst Biol Med 4: 565–583. |
1. | Angela Thach, Satu Kristiina Heiskanen, Brett L. Lucht, William B. Euler, The role of Zn2+-doping on the surface chemistry of poly(vinylidene difluoride) thin films, 2021, 23, 24680230, 101005, 10.1016/j.surfin.2021.101005 | |
2. | Navid Chapman, Mingyu Chapman, William B. Euler, Evolution of Surface Morphology of Spin-Coated Poly(Methyl Methacrylate) Thin Films, 2021, 13, 2073-4360, 2184, 10.3390/polym13132184 | |
3. | Jiangtao Xiang, Chaowei Hao, Qinghua Pan, Ze Li, Qingfang Ma, Investigation on the aggregation structure of poly(1-vinyl-2-pyrrolidone) concentrated solution in mixed solvents of water/N,N-dimethylformamide, 2023, 301, 0303-402X, 221, 10.1007/s00396-023-05057-6 | |
4. | E. D. Rychikhina, D. A. Semikov, Yu. I. Sachkov, A. I. Koptyaev, Continuous films of methyl pheophorbide a obtained by drop-casting from a multicomponent mixture for photoelectric measurements, 2023, 72, 1066-5285, 1542, 10.1007/s11172-023-3932-9 |
Property | THF | DMF |
Molecular weight (g/mol) | 72.1 | 73.1 |
Density (g/cm3) | 0.888 | 0.945 |
Molar volume (cm3/mol) | 81.08 | 77.43 |
Boiling point (℃) | 66 | 153 |
Vapor pressure (kPa, 21 ℃) | 17.7 | 0.507 |
Viscosity (mPa·s, 25 ℃) | 0.55 | 0.82 |
Surface tension (mN/m, 20 ℃) | 28 | 35 |