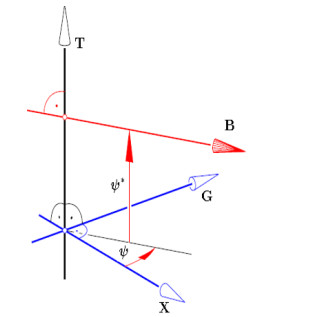
Citation: Nadia Alluhaibi. Ruled surfaces with constant Disteli-axis[J]. AIMS Mathematics, 2020, 5(6): 7678-7694. doi: 10.3934/math.2020491
[1] | Fatemah Mofarreh, Rashad A. Abdel-Baky . Spacelike ruled surfaces with stationary Disteli-axis. AIMS Mathematics, 2023, 8(4): 7840-7855. doi: 10.3934/math.2023394 |
[2] | Nadia Alluhaibi, Rashad A. Abdel-Baky . Kinematic-geometry of lines with special trajectories in spatial kinematics. AIMS Mathematics, 2023, 8(5): 10887-10904. doi: 10.3934/math.2023552 |
[3] | Awatif Al-Jedani, Rashad A. Abdel-Baky . One-parameter Lorentzian spatial kinematics and Disteli's formulae. AIMS Mathematics, 2023, 8(9): 20187-20200. doi: 10.3934/math.20231029 |
[4] | Yanlin Li, Ali. H. Alkhaldi, Akram Ali, R. A. Abdel-Baky, M. Khalifa Saad . Investigation of ruled surfaces and their singularities according to Blaschke frame in Euclidean 3-space. AIMS Mathematics, 2023, 8(6): 13875-13888. doi: 10.3934/math.2023709 |
[5] | Kemal Eren, Soley Ersoy, Mohammad Nazrul Islam Khan . Simultaneous characterizations of alternative partner-ruled surfaces. AIMS Mathematics, 2025, 10(4): 8891-8906. doi: 10.3934/math.2025407 |
[6] | Emad Solouma, Ibrahim Al-Dayel, Meraj Ali Khan, Youssef A. A. Lazer . Characterization of imbricate-ruled surfaces via rotation-minimizing Darboux frame in Minkowski 3-space E31. AIMS Mathematics, 2024, 9(5): 13028-13042. doi: 10.3934/math.2024635 |
[7] | Ayman Elsharkawy, Clemente Cesarano, Abdelrhman Tawfiq, Abdul Aziz Ismail . The non-linear Schrödinger equation associated with the soliton surfaces in Minkowski 3-space. AIMS Mathematics, 2022, 7(10): 17879-17893. doi: 10.3934/math.2022985 |
[8] | Emel Karaca . Non-null slant ruled surfaces and tangent bundle of pseudo-sphere. AIMS Mathematics, 2024, 9(8): 22842-22858. doi: 10.3934/math.20241111 |
[9] | Yanlin Li, Kemal Eren, Kebire Hilal Ayvacı, Soley Ersoy . Simultaneous characterizations of partner ruled surfaces using Flc frame. AIMS Mathematics, 2022, 7(11): 20213-20229. doi: 10.3934/math.20221106 |
[10] | Maryam T. Aldossary, Rashad A. Abdel-Baky . Sweeping surface due to rotation minimizing Darboux frame in Euclidean 3-space E3. AIMS Mathematics, 2023, 8(1): 447-462. doi: 10.3934/math.2023021 |
Line geometry possesses a close relation to spatial kinematics and has therefore found applications in mechanism design and robot kinematics. In spatial kinematics, it is interesting to study the intrinsic properties of the line trajectory from the concepts of ruled surface in differential geometry [1,2,3,4]. As it is known, dual numbers are used to investigate the motion of a line trajectory. Hence, the E. Study's map leads to the conclusion that the family of all directed lines in Euclidean three-space E3 is one-to- one correspondent with family of points of the dual unit sphere in the dual three- space D3 (see [1,2,3,6,9,10,11,12]).
This work offers an approach for constructing ruled surfaces with constant Disteli-axis by using E. Study map. Meanwhile, we determine kinematic- geometry of the Plücker conoid and its characterization. Finally, the well-known Dupin's indicatrix in surfaces theory has been defined for the kinematic geometry of the Plücker's conoid. Moreover, some characterizations equations for special ruled surfaces undrgoing one-parameter scrow motion are obtained and investigated. In addition, we have given some necessary and sufficient conditions to have constant dual angles with respect to constant Disteli-axis and we have discussed some special cases which lead to some special ruled surfaces such as the constant helicoids, One-sheeted circular hyperboloids, Archimedecs helicoids, circular cones and right helicoids.
Here, we give a brief introduction of some basic definitions and formulae that are associated to the topic (See for instance Refs. [6,10,11,12]). Recall that any directed line L in the Euclidean 3-space E3 has a representation in terms of a point p on L and a directed vector x of L such that ‖x‖=1. Therefore, the moment vector about the origin 0 in E3 can be given as
x∗=p×x. | (1) |
p can be replaced by any point
q=p+μx,μ∈R | (2) |
on L, then x∗ does not depend on the choice of p on line. Clearly, x and x∗ are dependent of each other. Moreover, we have
<x∗,x>=0,<x,x>=1. | (3) |
The components x∗i,xi(i=1,2,3) of x∗ and x are referred to as the normalized Plűcker coordinates of L. Therefore, L can be determined by the two vectors x and x∗.
The dual numbers set is
D={X=x+εx∗∣ x, x∗∈R, ε≠0, ε2=0}, | (4) |
which is a commutative ring. A dual number X=a+εx∗, is referred to as proper if x≠0. A dual angle formed by two skew lines in E3 gives an example of dual number in which it is given by Θ=ϑ+εϑ∗; where ϑ and ϑ∗ are the projected angle and the minimal distance between the lines, respectively.
Now, the set
D3={X=x+εx∗; (x,x∗)∈E3×E3 and ε≠0,ε2=0}, | (5) |
with the inner product
<X,Y>=<x,y>+ε(<y,x∗>+<y∗,x>), | (6) |
constructs the dual 3-space D3.Hence, a point X=(X1,X2,X3)t has dual coordinates Xi=(xi+εx∗i)∈D. The norm is given as
<X,X>12:=‖X‖=‖x‖(1+ε<x,x∗>‖x‖2). | (7) |
The set
K={X∈D3∣‖X‖2=X21+X22+X23=1}. | (8) |
gives the dual unit sphere in D3. All points X in K satisfy the two formulae
x21+x22+x23=1,x1x∗1+x2x∗2+x3x∗3=0. | (9) |
Therefore, E. Study's map ([6,10,11,12]) states that: There is a one to one map between the set of all oriented lines in E3 and the set of points of dual unit sphere in D3.
Definition 1. A family of oriented lines L=(a,a∗)∈E3 satisfying
C: <a∗,x>+<x∗,a>=0, | (10) |
where X=(x,x∗)∈E3 , is referred to as a line complex when <x,x∗>≠0 and is a singular (special) line complex when
<x∗,x>=0,<x,x>=1. | (11) |
Geometrically, a singular line complex is a family of all lines L=(a,a∗) intersecting the line X=(x,x∗) [6,10,11,12]. From E. Study's map, line complex can be defined using four independent parameters, so we can produce a finite number of lines, line congruence, given by intersecting any two line complexes. The intersection of two independent line congruences forms a differentiable set of lines in E3 defined as a ruled surface.
Ruled surfaces (such as cones and cylinders) contain generating lines in which the tangent plane touches the surface along the generator. Such generating lines are referred to as torsal lines:
∙ Ruled surfaces formed by only torsal generating line are known as developable surfaces;
∙ Ruled surfaces, in which most of its generators are non-torsal, are known as skew ruled surfaces;
∙ Ruled surfaces consisting of tangents of a spatial curve, , cones, and cylinders are developable surfaces.
One-parameter dual spherical motions
Let Km and Kf be two dual unit spheres with the common center O and two orthonormal dual coordinate frames {O; E1,E2,E3} and {O;F1,F2,F3}, respectively. Let us assume that the frame {O;F1,F2,F3} is fixed, and the set {O; E1,E2,E3} consists of real functions of parameter t (say the time). Then under assumption, Km moves about Kf and such motion is denoted by Km/Kf and referred to as a one-parameter dual spherical motion. If the line spaces Hm and Hf correspond to Km and Kf, respectively, then the one-parameter spatial motion Hm/Hf corresponds to Km/Kf. Therefore Hm moves about the fixed space Hf. Let A=(Aij) be a dual matrix. By putting <Fi,Ej>=Aij, the E. Study map can be written as [2,3,5]:
Km/Kf:(F1F2F3)=(A11A12A13A21A22A23A31A32A33)(E1E2E3). | (12) |
The dual matrix A:=(Aij)=a+εa∗=(aij)+ε(a∗ij) satisfies that A(u)A(u)t=I3, where I3 is the 3×3 identity matrix. Hence, detA(t)=1 and A(t) is an orthogonal dual matrix, . The Lie algebra L(OD3×3) of the group of positive orthogonal dual matrices A of rank 3×3 is the algebra of skew-symmetric dual matrices of rank 3×3
Ω(t)=A′At=( 0 Ω3−Ω2−Ω3 0 Ω1 Ω2−Ω1 0), |
where A′=dA(t)dt. Let us consider the following convention
Ω(t)=( 0 Ω3−Ω2−Ω3 0 Ω1 Ω2−Ω1 0)⇔(Ω1Ω2Ω3)=Ω. | (13) |
Consequently, we can consider the elements in L(OD3×3) as vectors of the usual 3-dimensional dual vector space or skew-symmeteric matrices. If dash refers to the derivation about t, then the derivative equation of the motion Km/Kf is:
(E′1E′2E′3)=( 0 Ω3−Ω2−Ω3 0 Ω1 Ω2−Ω1 0)(E1E2E3)=Ω×(E1E2E3). | (14) |
where \mathbf{\Omega }(t)\mathbf{ = \pmb{\mathsf{ ω}} +}\varepsilon \omega ^{\ast } = (\Omega _{1}, \Omega _{2}, \Omega _{3}) is known as the instantaneous dual rotation vector of \mathbb{K}_{m}/\mathbb{K}_{f}. \omega ^{\ast } and \omega , respectively, are real vectors corresponding to the instantaneous translational differential velocity vector and the instantaneous rotational differential velocity vector of the motion \mathbb{H}_{m}/\mathbb{H}_{f} [2,3,4,5].
During the motion \mathbb{H}_{m}/\mathbb{H}_{f} , every fixed line \mathbf{X} of the moving space \mathbb{H}_{m} , constructs a ruled surface in the fixed space \mathbb{H}_{f} denoted by (X) . In kinematics, such ruled surface is called line trajectory. To study the geometrical aspects of a line trajectory, we choose a moving frame, called the Blaschke frame, associated with the point on \mathbb{K}_{m} [2,3,4,5] as:
\begin{equation} \mathbf{X = X}(t), \ \mathbf{T}(t): = \mathbf{t}(t)\mathbf{+}\varepsilon \mathbf{t }^{\ast }(t) = \frac{\mathbf{X}^{^{\prime }}}{\left \Vert \mathbf{X}^{^{\prime }}\right \Vert }, \; \mathbf{G}(t): = \mathbf{g}(t)\mathbf{+}\varepsilon \mathbf{g }^{\ast }(t) = \mathbf{X}\times \mathbf{T}. \end{equation} | (15) |
\mathbf{X} , \mathbf{T} , and \mathbf{G} are three dual unit vectors corresponding to three concurrent mutually perpendicular lines \mathbb{E}^{3} and they intersect at a point \mathbf{C} on \mathbf{X} called central point. \mathbf{G}(t) is the limit position of the shared perpendicular to \mathbf{X}(t) and \mathbf{X}(t+dt) , and is referred to as the central tangent of (X) at \mathbf{C} . All central points form the striction curve. The vector \mathbf{T} is known as the central normal of \mathbf{X} at \mathbf{C} . By construction, the Blaschke motion \mathbb{K}_{m}/\mathbb{K }_{f}\; is given by
\begin{equation} \mathbb{K}_{m}/\mathbb{K}_{f}:\left( \begin{array}{l} \mathbf{X}^{^{\prime }} \\ \mathbf{T}^{^{\prime }} \\ \mathbf{G}^{^{\prime }} \end{array} \right) = \left( \begin{array}{lll} \ \ 0 &\ P &\ 0 \\ -P &\ \ 0 & Q \\ \ \ 0 & -Q &\ 0 \end{array} \right) \left( \begin{array}{l} \mathbf{X} \\ \mathbf{T} \\ \mathbf{G} \end{array} \right) = \mathbf{\Omega \times }\left( \begin{array}{l} \mathbf{X} \\ \mathbf{T} \\ \mathbf{G} \end{array} \right) , \end{equation} | (16) |
where
\begin{equation} P = p+\varepsilon p^{\ast } = \left \Vert \mathbf{X}^{^{\prime }}\right \Vert , \;Q: = q+\varepsilon q^{\ast } = \det (\mathbf{X, X}^{^{\prime }}, \mathbf{X} ^{^{^{\prime \prime }}})\left \Vert \mathbf{X}^{^{\prime }}\right \Vert ^{^{-}2}, \end{equation} | (17) |
are called the Blaschke invariants of (X) , and \mathbf{ \Omega \mathbf{(}}t\mathbf{\mathbf{)} = }Q\mathbf{X+}P\mathbf{G} . The tangent of the striction curve \mathbf{C} may be written as
\begin{equation} \mathbf{C}^{^{\prime }} = q^{\ast }\mathbf{x}+p^{\ast }\mathbf{g.} \end{equation} | (18) |
Furthermore, the distribution parameters of the surfaces (X) , (T) , and (G) , respectively, are:
\begin{equation} \mu (t) = \frac{p^{\ast }}{p}\text{, }\delta (t) = \frac{pp^{\ast }+qq^{\ast }}{ q^{2}+p^{2}}\text{, and }\Gamma (t) = \frac{q^{\ast }}{q}. \end{equation} | (19) |
Now,
\begin{equation} \mathbf{B\mathbf{(}}t\mathbf{\mathbf{)} = \mathbf{b}(}t)+\mathbf{ \pmb{\mathsf{ ε}} \mathbf{b}^{\ast }(}t\mathbf{) = \frac{\mathbf{\Omega }}{\left \Vert \mathbf{ \Omega }\right \Vert } = }\frac{Q\mathbf{X+}P\mathbf{G}}{\sqrt{Q^{2}+P^{2}}}, \end{equation} | (20) |
is a dual unit vector such that \Omega : = \omega +\varepsilon \omega ^{\ast } = \left \Vert \mathbf{\Omega } \right \Vert is referred to as dual angular speed of the Blaschke frame. Clearly, \mathbf{B} is the Disteli-axis (striction axis or evolute) of (X) . For each generating line of a cylindrical surface, the Disteli-axis and the curvature axis of the perpendicular sections are identified. From Eq (20), the Disteli-axis is perpendicular to the central normal \mathbf{T} , and is parallel to the tangent plane of (X) . Let \Psi = \psi +\varepsilon \psi ^{\ast } be a dual angle between \mathbf{B} and \mathbf{X} , then we have that:
\begin{equation} \mathbf{B}(t) = \cos \Psi \mathbf{X+}\sin \Psi \mathbf{G}, \end{equation} | (21) |
where
\begin{equation} \cot \Psi = \frac{Q}{P}. \end{equation} | (22) |
Note that \Psi is the dual spherical radius of curvature. The trigonometric function in Eq (22) of \Psi can be expanded as:
\begin{equation} \cot \Psi = \cot \psi -\varepsilon \psi ^{\ast }(1+\coth ^{2}\psi ) = \frac{ q+\varepsilon q^{\ast }}{p+\varepsilon p^{\ast }}. \end{equation} | (23) |
One of the invariants of the dual curve \mathbf{X}(t) on \mathbb{K}_{f} is
\begin{equation} \Sigma (t): = \gamma +\varepsilon \left( \Gamma -\mu \gamma \right) = \frac{Q}{P }, \end{equation} | (24) |
called the dual geodesic curvature. Here \gamma (t) = \frac{q}{p} is the geodesic curvature of the spherical image curve t\in I\mapsto \mathbf{x(}t) of (X) . Hence, from the dual and real parts of Eqs (23) and (24), respectively, we get
\begin{equation} \psi ^{\ast }(t) = \frac{1}{2}\left( \mu -\Gamma \right) \sin 2\psi, \end{equation} | (25) |
and
\begin{equation} \gamma (t) = \cot \psi = \frac{q}{p}. \end{equation} | (26) |
Here, \psi ^{\ast } is the normal distance from \mathbf{X} to the Distelli-axis \mathbf{B} along the central tangent of (X) . The functions \Gamma (t) , \mu (t) and \gamma (t) are referred to as construction parameters of (X) or the curvature functions.
We now are interesting to research kinematics and the geometrical properties of the line trajectory (X) . So, we are going to do a detailed study of the Blaschke invariants P(t) , and Q(t) . To carry out this, from Eq (16), we can write the following equations:
\begin{equation} \mathbf{X}^{^{^{\prime }}}(t) = \left( \left \Vert \mathbf{\Omega }\right \Vert \mathbf{B}\right) \times \mathbf{X}\text{, }\mathbf{T}^{^{^{\prime }}}(t) = \left( \left \Vert \mathbf{\Omega }\right \Vert \mathbf{B}\right) \times \mathbf{T}\text{, }\mathbf{G}^{^{^{\prime }}}(t) = \left( \left \Vert \mathbf{\Omega }\right \Vert \mathbf{B}\right) \times \mathbf{G.} \end{equation} | (27) |
Hence, at any instant, it is seen that: \left \Vert \mathbf{\Omega }\right \Vert = \Omega = \omega +\varepsilon \omega ^{\ast } is the dual angular speed of the motion \mathbb{K}_{m}/\mathbb{K}_{f} about \mathbf{B} . The parts
\begin{equation} \omega ^{\ast }(t) = \frac{pp^{\ast }+qq^{\ast }}{\sqrt{q^{2}+p^{2}}}, \text{ and }\omega (t) = \sqrt{p^{2}+q^{2}}, \end{equation} | (28) |
corresponding to translational angular speed and the rotational angular speed of the motion \mathbb{H}_{m}/\mathbb{H}_{f} along \mathbf{B} , respectively.
Hence, we have the following corollary:
Corollary 1. For the one-parameter Blaschke motion, at any instant t , the pitch of the motion h(t) can be given by
\begin{equation} h(t): = \frac{ \lt \mathbf{ \pmb{\mathsf{ ω}} , \pmb{\mathsf{ ω}} }^{\ast }\mathbf{ \gt }}{\left \Vert \mathbf{ \pmb{\mathsf{ ω}} }\right \Vert ^{2}} = \mu \cos ^{2}\psi +\Gamma \sin ^{2}\psi . \end{equation} | (29) |
Now, assume that the dual vector \mathbf{\Omega = \pmb{\mathsf{ ω}} +} \varepsilon \omega ^{\ast } is given, then the list of the following physical properties of the Blaschke motion can be determined:
(i) The Disteli-axis \mathbf{B} can be represented by Eq (20).
(ii) The dual angular speed of its dual angular velocity can be expressed as \left \Vert \mathbf{\Omega }\right \Vert = \omega (1+\varepsilon h) .
(iii) If \mathbf{y} is a point on the Disteli-axis \mathbf{B} , then we get:
\begin{equation} \mathbf{y}(t, v)\mathbf{ = b}\times \mathbf{b}^{\ast }+v\mathbf{b}, \;v\in \mathbb{R}. \end{equation} | (30) |
This parametrization defines a non-developable ruled surface (B) . If h(u) = 0 , i.e., the Blaschke motion \ is pure rotation, then
\begin{equation} \mathbf{B}(t)\mathbf{ = b}(t)+\varepsilon \mathbf{b}^{\ast }(t) = \frac{1}{\left \Vert \omega \right \Vert }(\omega +\varepsilon \omega ^{\ast }), \end{equation} | (31) |
whereas if h(t) = 0 , and \left \Vert \omega \right \Vert ^{2} = 1 , then \mathbf{\Omega } is an directed line. However, if \mathbf{\Omega = } 0 \mathbf{+}\varepsilon \omega ^{\ast } , i.e., of the Blaschke motion \ is pure translational, we put \omega ^{\ast } = \left \Vert \mathbf{\Omega }^{\ast }\right \Vert; \omega ^{\ast } \mathbf{b = }\boldsymbol{\omega }^{\ast } for arbitrary \mathbf{b} ^{\ast } such that \omega ^{\ast }\neq 0 , otherwise \mathbf{b} can be arbitrarily chosen, too.
The Eqs in (26) and (29), respectively, are resemblances to the Mannhiem and Hamilton formulae of surfaces theory in Euclidean 3-space. Now we intend to give geometrical interpretations of these formulae. Next, the parametric representation of \psi ^{\ast } in Eq (26) is the well known Plücker's conoid, or cylindroid. The Plücker conoid is a differentiable regular ruled surface [6,10,11]. The parametric representation can also be given by using point coordinates. To do that, let \mathbf{T} and y- axis of a fixed Euclidean space frame (oxyz) be coincident and the position of the dual unit vector \mathbf{B} be determined by angle \psi and distance \psi ^{\ast } along the positive direction of y- axis. The dual unit vectors \mathbf{X} and \mathbf{G} can be chosen in terms of x and z -axes, respectively. This shows that the dual unit vectors \mathbf{X} and \mathbf{G} together with \mathbf{T} form the coordinate system of the Plücker's conoid, as depicted in Figure 1. Let \mathbf{y} be a point on the surface, then we have the point coordinates
\begin{equation} M:\mathbf{y}(t, v)\mathbf{ = }(0, \psi ^{\ast }, 0)+v(\cos \psi , 0, \sin \psi ), \ \ v\in \mathbb{R} , \end{equation} | (32) |
Using such representation, the generator \mathbf{B} is clearly visible passing through the y- axis. Thus,
\begin{equation} \psi ^{\ast }: = y = \frac{1}{2}\left( \mu -\Gamma \right) \sin 2\psi , \text{ } x = v\cos \psi \text{, and }z = v\sin \psi , \end{equation} | (33) |
where \psi ^{\ast } gives the point of the intersection of the principal axes \mathbf{X} and \mathbf{G} which locates at a half of the conoid height. By direct calculations,
\begin{equation} \left( y^{2}+z^{2}\right) y+\left( \Gamma -\mu \right) zy = 0, \end{equation} | (34) |
which is the algebraic equation for Plücker's conoid. The Plücker's conoid described by Eq (34) depends only on the difference of its two integral invariants of the first order; \Gamma -\mu = 1, \ 0\leq \psi \leq 2\pi , -4\leq \upsilon \leq 4 (Figure 2). Furthermore, one can get a second-order equation in \frac{y }{z} in which its solutions are given by
\begin{equation} \frac{y}{z} = \frac{1}{2y}\left[ \mu -\Gamma \pm \sqrt{\left( \Gamma -\mu \right) ^{2}-4y^{2}}\right] . \end{equation} | (35) |
By equating the discriminant of Eq (35) to zero, we get the limits of the Plücker's conoid. Hence, the two extreme positions are given by,
\begin{equation} y = \pm \left( \Gamma -\mu \right) /2. \end{equation} | (36) |
Equation (36) gives the positions of the two torsal planes, each of which contains one torsal line L .
On the other hand, h(u) in Eq (29) is periodic function and to have at most two extreme values, the curvature functions \mu and \Gamma . Therefore, the directed lines \mathbf{X} and \mathbf{G} are principal axes of the Plücker's conoid. However, the geometric aspects of the Plü cker's conoid are discussed as follows:
(i)- If h(t)\neq 0 , then we have two real generating lines moving through the point (0, y, 0) if y < \left(\Gamma -\mu \right) /2 ; and for the two limit points y = \pm \left(\Gamma -\mu \right) /2 , the generators and the principal axes \mathbf{X} and \mathbf{G} are coincident.
(ii)- If h(t) = 0, then the two torsal lines L_{1} , L_{2} are represented by
\begin{equation} L_{1}, \ L_{2}:\ \frac{x}{z} = \cot \psi = \pm \sqrt{-\frac{\mu }{\Gamma }}, ~y = \pm \left( \Gamma -\mu \right) /2, \end{equation} | (37) |
which shows that the two torsal lines L_{1}, and \ L_{2} are perpendicular each other. So, if \mu and \Gamma are equal, then the Plücker's conoid becomes pencil of lines through the origin " \mathbf{o"} in the torsal plane y = 0 . In this case L_{1} and L_{2} are the principal axes of an elliptic line congruence. However, if \mu and \Gamma have opposite signs, then L_{1} and L_{2} are real and to be coincident with the principal axes of a hyperbolic line congruence. If either \mu or \Gamma is zero then the lines L_{1} and L_{2} both coincide with y- axis; for \mu \neq 0 , \Gamma = 0 or for \Gamma \neq 0, \mu = 0 they coincide with z- axis.
Furthermore, to convert from polar coordinates to Cartesian, we use the substitution
\begin{equation*} y = \frac{\cos \psi }{\sqrt{h}}\text{, }z = \frac{\sin \psi }{\sqrt{h}}, \end{equation*} |
in Hamilton's formula, one obtain the equation
\begin{equation*} D:\left \vert \mu \right \vert y^{2}+\left \vert \Gamma \right \vert z^{2} = 1, \end{equation*} |
of a conic section. This conic section is the Dupin's indicatrix of the Pl ücker's conoid. We now examine three cases in detail:
(1) If \mu , and \Gamma are both positive, the Dupin's indicatrix is an ellipse has the principal semi-axes are \frac{1}{\sqrt{\mu }} , and \frac{1 }{\sqrt{\Gamma }} . The lines through the center intersects the ellipse in the points:
\begin{equation*} y = \pm \frac{\cos \psi }{\sqrt{h}}\text{, }z = \pm \frac{\sin \psi }{\sqrt{h}}. \end{equation*} |
The distance intercepted by the ellipse on the line \frac{z}{y} = \tan \psi is:
\begin{equation*} \sqrt{y^{2}+z^{2}} = \frac{1}{\sqrt{h}}. \end{equation*} |
(2) If \mu , and \Gamma have opposite signs the Dupin's indicatrix is set of conjugate hyperbolas
\begin{equation*} D:\mu x^{2}\mp \Gamma y^{2} = \pm 1. \end{equation*} |
The two asymptotic directions of the hyperbolas represent the torsal lines at which h = 0 ;
(3) If either \mu or \Gamma is zero the Dupin's indicatrix is a set of parallels lines corresponding to one of the forms:
\begin{equation*} z^{2} = \left \vert \frac{1}{\Gamma }\right \vert \text{ with }\mu = 0\text{, or }y^{2} = \left \vert \frac{1}{\mu }\right \vert \text{ with }\Gamma = 0. \end{equation*} |
Serret–Frenet motion
If p^{\ast } = 0 in Eq (18), then (X) is a tangential developable ruled surface, that is, \mathbf{C}^{^{^{\prime }}} = \mathbf{x} . In this case, the Blaschke frame \{ \mathbf{x}, \mathbf{t} , \mathbf{g}\} coincides with the classic Serret–Frenet frame and then the striction curve \mathbf{C} becomes the edge of regression of (X) . Hence, p and q are the curvature \kappa , and the torsion \tau of \mathbf{C} , respectively. Moreover, q^{\ast } = 1 , and \Gamma = 1/\tau . Also \Gamma is the radius of torsion of \mathbf{C} . By similar argument, we can also have the following equations:
\begin{equation} h(u) = \frac{\tau }{\tau ^{2}+\kappa ^{2}} = \frac{1}{\tau }\sin ^{2}\psi \text{ , }\; \; \psi ^{\ast } = \frac{1}{2\tau }\sin 2\psi , \; \; \text{ with }\tan \psi = \frac{ \tau }{\kappa }. \end{equation} | (38) |
The corresponding Plücker conoid is
\begin{equation} M:\mathbf{ \pmb{\mathsf{ ζ}} }(t, v)\mathbf{ = }(0, \psi ^{\ast }, 0)+v(\cos \psi , 0, \sin \psi ), \ \ \upsilon \in \mathbb{R}. \end{equation} | (39) |
This yields
\begin{equation} \left( z^{2}+y^{2}\right) y+\Gamma zy = 0. \end{equation} | (40) |
In the following, when we say (X) is a constant Disteli-axis, we mean that all the generators of the ruled surface (X) have a constant dual angle from its Disteli-axis. The dual arc length d\widehat{s} = ds+\varepsilon ds^{\ast } of \mathbf{X}(t) is
\begin{equation} \widehat{s}(t) = \overset{u}{\underset{0}{\int }}Pdt = \overset{u}{\underset{0}{ \int }}p(1+\varepsilon \mu )dt. \end{equation} | (41) |
Now, we use the dual arc length parameter \widehat{s} instead of t . If the prime means to differentiate with respect to \widehat{s} , then from Eq (16), we get
\begin{equation} \left( \begin{array}{l} \mathbf{X}^{^{\prime }} \\ \mathbf{T}^{^{\prime }} \\ \mathbf{G}^{^{\prime }} \end{array} \right) = \left( \begin{array}{lll} 0 & 1 & 0 \\ -1 & 0 & \Sigma \\ 0 & -\Sigma & 0 \end{array} \right) \left( \begin{array}{l} \mathbf{X} \\ \mathbf{T} \\ \mathbf{G} \end{array} \right) = \mathbf{\Omega \times }\left( \begin{array}{l} \mathbf{X} \\ \mathbf{T} \\ \mathbf{G} \end{array} \right) , \end{equation} | (42) |
where \mathbf{\Omega = \Sigma X+G} . Thus, we may write the following relationships:
\begin{equation} \widehat{\kappa }: = \kappa +\varepsilon \kappa ^{\ast } = \sqrt{1+\Sigma ^{2}} = \sin \Psi = \frac{1}{\widetilde{\rho }}\text{, }\widehat{\tau }: = \tau +\varepsilon \tau ^{\ast } = \pm \frac{\Sigma ^{^{\prime }}}{\sqrt{1+\Sigma ^{2}}} = \pm \Psi ^{^{\prime }}. \end{equation} | (43) |
Here, \ \widehat{\kappa } and \widehat{\tau } refer to the dual curvature function and the dual torsion function of \mathbf{X} = \mathbf{X}(\widehat{s}) , respectively. The terms found in Eq (43) are analogous to their counterparts in 3-dimensional Euclidean spherical geometry.
Definition 2. For one-parameter Blaschke motion, at the instant \widehat{s}\in \mathbb{D} , a directed line \mathbf{Y} in fixed space is said to be \mathbf{B}_{k} -Disteli-axis of (X)
\left. \begin{array}{l} \lt {\bf{Y}},{{\bf{X}}^i}(\hat s) \gt = 0\;,\;\;1 \le i \le k\\ \lt {\bf{Y}},{{\bf{X}}^{k + 1}}(\hat s) \gt \ne 0. \end{array} \right\} |
Here \mathbf{X}^{i} refers to the i-th differentiation of \mathbf{X} .
Based on above, consider
\begin{equation} \widetilde{\rho } = \cos ^{-1}\left( \lt \mathbf{Y, X \gt }\right) \mathbf{, } \end{equation} | (44) |
which a dual angle such that \mathbf{Y} , and \widetilde{\rho } remain constant up to the second order at \widehat{s} = \widehat{s}_{0} , hence
\begin{equation} \widetilde{\rho }^{^{\prime }}\mid \widehat{s} = \widehat{s}_{0} = 0, \; \; \mathbf{X}^{^{\prime }}\mid \widehat{s} = \widehat{s}_{0} = \mathbf{0}, \end{equation} | (45) |
and
\begin{equation*} \widetilde{\rho }^{^{^{\prime \prime }}}\mid \widehat{s} = \widehat{s}_{0} = 0, \; \mathbf{X}^{^{^{\prime \prime }}}\mid \widehat{s} = \widehat{s}_{0} = \mathbf{0}. \end{equation*} |
Therefore,
\begin{equation} \lt \mathbf{X}^{^{\prime }}, \mathbf{Y \gt } = 0, \end{equation} | (46) |
and
\begin{equation*} \lt \mathbf{X}^{^{^{\prime \prime }}}, \mathbf{Y \gt } = 0. \end{equation*} |
Then, \widetilde{\rho } is invariant in the second approximation if and only if \mathbf{Y} is the Disteli-axis \mathbf{B} of (X) , i.e.,
\begin{equation} \widetilde{\rho }^{^{\prime }} = \widetilde{\rho }^{^{\prime \prime }} = 0\Leftrightarrow \mathbf{Y\mathbf{ = }\frac{\mathbf{X}^{^{\prime }}\times \mathbf{X}^{^{\prime \prime }}}{\left \Vert \mathbf{X}^{^{\prime }}\times \mathbf{X}^{^{\prime \prime }}\right \Vert } = }\pm \mathbf{B}\text{.} \end{equation} | (47) |
Now, we shall act as we have done in the last. Using the definition of the Disteli-axis, we get the dual frame;
\begin{equation} \mathbf{U}_{1} = \mathbf{B}(\widehat{s}) , \ \mathbf{U}_{2}(\widehat{s}) = \frac{\mathbf{B}^{^{\prime }}}{\left \Vert \mathbf{B}^{^{\prime }}\right \Vert }, \ \mathbf{U}_{3}(\widehat{s}) = \mathbf{B}\times \mathbf{U}_{2}, \end{equation} | (48) |
as the Blaschke frame along \mathbf{B}(t) . Thus, the calculations give that:
\begin{equation} \left( \begin{array}{l} \mathbf{U}_{1} \\ \mathbf{U}_{2} \\ \mathbf{U}_{3} \end{array} \right) = \left( \begin{array}{lll} \cos \Psi & 0 & \sin \Psi \\ -\sin \Psi & 0 & \cos \Psi \\ \ \ \ \ \ 0 & -1 & \ \ \ \ \ 0 \end{array} \right) \left( \begin{array}{l} \mathbf{X} \\ \mathbf{T} \\ \mathbf{G} \end{array} \right) . \end{equation} | (49) |
The variations of this frame are analogous to Eqs. (16) and is given by:
\begin{equation} \left( \begin{array}{l} \mathbf{U}_{1}^{^{\prime }} \\ \mathbf{U}_{2}^{^{\prime }} \\ \mathbf{U}_{3}^{^{\prime }} \end{array} \right) = \left( \begin{array}{lll} \ \text{ }0 & \ \ \Psi ^{^{\prime }} & 0 \\ -\Psi ^{^{\prime }} & \ \ 0 & \widehat{\kappa } \\ \text{ }\ 0 & -\widehat{\kappa } & \ 0 \end{array} \right) \left( \begin{array}{l} \mathbf{U}_{1} \\ \mathbf{U}_{2} \\ \mathbf{U}_{3} \end{array} \right) = \widetilde{\mathbf{\Omega }}\mathbf{\times }\left( \begin{array}{l} \mathbf{U}_{1} \\ \mathbf{U}_{2} \\ \mathbf{U}_{3} \end{array} \right) , \end{equation} | (50) |
where \widetilde{\mathbf{\Omega }}(\widehat{s})\mathbf{ = }\widehat{\kappa } \mathbf{U}_{1}+\Psi ^{^{\prime }}\mathbf{U}_{3} is the Darboux vector. From Eqs (42) and (50), we obtain that:
\begin{equation} \mathbf{\Omega }-\widetilde{\mathbf{\Omega }} = -\Psi ^{^{\prime }}\mathbf{T.} \end{equation} | (51) |
From Eqs (43) and (51), we obtain that: If T(t) = \tau +\varepsilon \tau ^{\ast } = 0 ( \Sigma ^{^{\prime }} = 0 ), i.e. \psi and \psi ^{\ast } are constants, then the line \mathbf{X} moves about it with constant pitch h and the Disteli-axis is fixed to the second order. Therefore, the surface (X) is locally traced during a one-parameter screw motion with respect to the constant Disteli-axis \mathbf{B} , by the line \mathbf{X} positioned at a constant distance \psi ^{\ast } and constant angle \psi relative to \mathbf{B} . Consequently, we have:
Theorem 1. Any non-developable ruled surface ( X ) is a constant Disteli-axis if and only if (a) \gamma = constant, and (b) \Gamma -\mu \gamma = constant.
We will now construct ruled surfaces with the constant Disteli-axis. From Eq (42), we have the following ordinary dual differential equation
\begin{equation} \mathbf{X}^{^{^{\prime \prime \prime }}}+\widetilde{\kappa }^{2}\mathbf{X} ^{\prime } = \mathbf{0}. \end{equation} | (52) |
Case (I). If \Sigma \neq 0 , then without loss of generality, we may assume \mathbf{X}^{^{\prime }}(0)\mathbf{ = }(0, 1, 0) . Under such initial condition, a dual unit vector \mathbf{X}^{^{\prime }} is given as
\begin{equation*} \mathbf{X}^{^{\prime }}(\widehat{s})\mathbf{ = }A_{1}\sin \left( \widetilde{ \kappa }\widehat{s}\right) \mathbf{F}_{1}+\left( \cos \left( \widetilde{ \kappa }\widehat{s}\right) +A_{2}\sin \left( \widetilde{\kappa }\widehat{s} \right) \right) \mathbf{F}_{2}+A_{3}\sin \left( \widetilde{\kappa }\widehat{s }\right) \mathbf{F}_{3}, \end{equation*} |
where A_{1} , A_{2} , and A_{3} are some dual constants satisfying A_{1}^{2}+A_{3}^{2} = 1 , and A_{2} = 0 . From this, we can obtain
\begin{equation*} \mathbf{X}(\widehat{s})\mathbf{ = }\left( -\widetilde{\rho }A_{1}\cos \left( \widetilde{\kappa }\widehat{s}\right) +D_{1}\right) \mathbf{F}_{1}+ \widetilde{\rho }\sin \left( \widetilde{\kappa }\widehat{s}\right) \mathbf{F} _{2}+\left( -\widetilde{\rho }A_{3}\cos \left( \widetilde{\kappa }\widehat{s} \right) +D_{3}\right) \mathbf{F}_{3}, \end{equation*} |
where D_{1} , D_{3} , are some dual constants satisfying A_{1}D_{1}+A_{3}D_{3} = 0, and D_{1}^{2}+D_{3}^{2} = 1 . If we adopt the dual coordinates transformation such that
\begin{equation*} \left( \begin{array}{l} \overline{X_{1}} \\ \overline{X_{2}} \\ \overline{X_{3}} \end{array} \right) = \left( \begin{array}{lll} A_{1} & 0 & A_{3} \\ 0 & 1 & 0 \\ -A_{3} & 0 & A_{1} \end{array} \right) \left( \begin{array}{l} X_{1} \\ X_{2} \\ X_{3} \end{array} \right) , \end{equation*} |
with respect to the new coordinates \overline{X_{i}} , the dual vector \mathbf{X}(\widehat{s}) becomes
\begin{equation} \mathbf{X}(\widehat{s})\mathbf{ = }-\widetilde{\rho }\cos \left( \widetilde{ \kappa }\widehat{s}\right) \mathbf{F}_{1}\mathbf{+}\widetilde{\rho }\sin \left( \widetilde{\kappa }\widehat{s}\right) \mathbf{F}_{2}+D\mathbf{F}_{3}, \end{equation} | (53) |
for a dual constant D = A_{1}D_{3}-A_{3}D_{3} , with D = \pm \cos \Psi . It is noted that \mathbf{X}(\widehat{s}) does not depend on the choice of the upper or lower sign of \pm . Hence, throughout the study we choose upper sign, that is,
\begin{equation} \mathbf{X}(\Phi )\mathbf{ = }-\cos \Phi\sin \Psi \mathbf{F}_{1}\mathbf{+} \sin \Phi\sin \Psi \mathbf{F}_{2}+\cos \Psi \mathbf{F}_{3}. \end{equation} | (54) |
where \Phi = \varphi +\varepsilon \varphi ^{\ast } = \widetilde{\kappa } \widehat{s} . This means that the lines \mathbf{B} and \mathbf{F}_{3} are coincident, and
\begin{equation} \psi = c_{1}(\text{real}\;const.), \; \psi ^{\ast } = c_{2}(\text{real}\;const.). \end{equation} | (55) |
Since \varphi , and \varphi ^{\ast } are two-independent parameters, the surface (X) is a line congruence \mathbb{E}^{3} .
Now, the Plücker coordinates can be used to determine the equation of this line congruence. Computing the dual and real parts of Eq (54), we get
\begin{equation} \mathbf{x}^{\ast }(\varphi , \varphi ^{\ast }) = \left( \begin{array}{c} x_{1}^{\ast } \\ x_{2}^{\ast } \\ x_{3}^{\ast } \end{array} \right) = \left( \begin{array}{l} -\psi ^{\ast }\cos \psi \cos \varphi +\varphi ^{\ast }\sin \psi \sin \varphi \\ \psi ^{\ast }\cos \psi \sin \varphi +\varphi ^{\ast }\sin \psi \cos \varphi \\ \ \ \ \ \ \ \ \ \ \ \ \ \ \ \ -\psi ^{\ast }\sin \psi \end{array} \right) . \end{equation} | (56) |
and
\begin{equation} \mathbf{x}(\varphi )\mathbf{ = }\left( -\cos \varphi \sin \psi , \sin \varphi\sin \psi , \cos \psi \right) , \end{equation} | (57) |
Let \mathbf{r}(r_{1}, r_{2}, r_{3}) be a point on the line \mathbf{X} . Since \mathbf{r\times x} = \mathbf{x}^{\ast } , we get a system of linear equations in r_{1}, r_{2}, and r_{3} :
\left. \begin{array}{l} - {r_3}\sin \varphi \sin \psi + {r_2}\cos \psi = x_1^ * ,\\ - {r_3}\cos \varphi \sin \psi - {r_1}\cos \psi = x_2^ * ,\\ {r_1}\sin \varphi \sin \psi + {r_2}\cos \varphi \sin \psi = x_3^ * . \end{array} \right\} |
The matrix of coefficients of unknowns r_{1}, r_{2}, and r_{3} is the skew 3\times 3 matrix
\begin{equation*} \left( \begin{array}{lll} \ \ \ \ 0 & \cos \psi & -\sin \psi \sin \varphi \\ -\cos \psi & \ \ \ \ \ \ 0 & -\sin \psi \cos \varphi \\ \sin \psi \sin \varphi & \sin \psi \cos \varphi & \ \ \ \ \ \ \ \ \ 0 \end{array} \right) , \end{equation*} |
and therefore its rank is 2 with \psi \neq 0 , and \varphi \neq 0 . Moreover, the rank of the augmented matrix
\begin{equation*} \left( \begin{array}{lllc} \ \ \ \ 0 & \cos \psi & -\sin \psi\sin \varphi & x_{1}^{\ast } \\ -\cos \psi & \ \ \ \ \ \ 0 & -\sin \psi\cos \varphi & x_{2}^{\ast } \\ \sin \psi \sin \varphi & \sin \psi\cos \varphi & \ \ \ \ \ \ \ \ \ 0 & x_{3}^{\ast } \end{array} \right) , \end{equation*} |
equals 2. Therefore, the system has infinitely many solutions represented by
\left. \begin{array}{l}-r_{1} = \left( r_{3}+\varphi ^{\ast }\right) \cos \varphi \tan \psi +\psi ^{\ast }\sin \varphi , \\ r_{2} = \left( r_{3}+\varphi ^{\ast }\right) \sin \varphi \tan \psi -\psi ^{\ast }\cos \varphi , \\ r_{1}\sin \psi \sin \varphi +r_{2}\sin \psi \cos \varphi = x_{3}^{\ast }.\end{array} \right\} | (58) |
Since r_{3} is taken at random, then we may take r_{3}+\varphi ^{\ast } = 0 . Then, Eq (58) becomes
\left. \begin{array}{l}r_{1}(t) = -\psi ^{\ast }\sin \varphi , \\ r_{2}(t) = -\psi ^{\ast }\cos \varphi , \\ r_{3}(t) = -\varphi ^{\ast }.\end{array} \right\} | (59) |
Let \mathbf{y} be a point on such line congruence. According to Eq (2), we get
\begin{equation} \mathbf{y}(\varphi , \varphi ^{\ast }, v) = \mathbf{r}(\varphi , \varphi ^{\ast })+v\mathbf{x(}\varphi , \varphi ^{\ast }\mathbf{), }\text{ }v\in \mathbb{R}. \end{equation} | (60) |
By means of Eqs (57) and (59) we obtain:
\begin{equation} \mathbf{y}(\varphi , \varphi ^{\ast }, v) = \left( \begin{array}{l} -\psi ^{\ast }\sin \varphi -v\cos \varphi \sin \psi \\ -\psi ^{\ast }\cos \varphi +v\sin \varphi \sin \psi \\ -\varphi ^{\ast }+v\cos \psi \end{array} \right) , \end{equation} | (61) |
which contains the family of ruled surfaces \mathbf{y}(\varphi _{0}, \varphi ^{\ast }, v) , \mathbf{y}(\varphi, \varphi _{0}^{\ast }, v) , and \mathbf{y}(\varphi (t), \varphi ^{\ast }(t), v) . Here \varphi _{0}^{\ast } , \varphi _{0} , and t are real constants. Let (x, \; y, \; z) be coordinates of \mathbf{y} , then Eq (61) yield
\left. \begin{array}{l}x = -\psi ^{\ast }\sin \varphi -v\cos \varphi \sin \psi , \\ y = -\psi ^{\ast }\cos \varphi +v\sin \varphi \sin \psi , \\ z = -\varphi ^{\ast }+v\cos \psi ,\end{array} \right\} | (62) |
By eliminating the parameter \varphi , we get
\begin{equation} (X):\frac{x^{2}}{\psi ^{\ast 2}}+\frac{y^{2}}{\psi ^{\ast 2}}-\frac{Z^{2}}{ k^{2}} = 1, \end{equation} | (63) |
where k = \psi ^{\ast }\cot \psi and Z = z+\varphi ^{\ast } . Then (X) is a one-parameter set of one-sheeted hyperboloids. The intersection of every hyperboloid and its corresponding plane z+\varphi ^{\ast } = 0 is the circular cylinder y^{2}+x^{2} = \psi ^{\ast 2} . Therefore the envelope of (X) is the cylinder y^{2}+x^{2} = \psi ^{\ast 2} . Notice that if \varphi ^{\ast } = 0 , then
\begin{equation} (X):\frac{x^{2}}{\psi ^{\ast 2}}+\frac{y^{2}}{\psi ^{\ast 2}}-\frac{z^{2}}{ \left( \psi ^{\ast }\coth \psi \right) ^{2}} = 1. \end{equation} | (64) |
Constant parameter ruled surfaces
A correlation between the two parameters \varphi and \varphi^{\ast } such as f(\varphi, \varphi^{\ast }) = 0 reduces the line congruence Eq (54) (resp. (61)) to a one-parameter set of lines (a ruled surface). So, if we set \varphi ^{\ast } = h\varphi in which h and \varphi are the pitch and the parameter of the motion \mathbb{H}_{m}/ \mathbb{H}_{f} , resp., then Eq (54) (resp. (61)) gives a ruled surface in \mathbb{H}_{f}- space. Hence we get the following relation:
\begin{equation*} \left( \begin{array}{l} \mathbf{X} \\ \mathbf{T} \\ \mathbf{G} \end{array} \right) = \left( \begin{array}{lll} -\sin \Psi \cos \Phi & \sin \Psi \sin \Phi & \cos \Psi \\ \ \ \ \ \ \sin \Phi & \cos \Phi & 0 \\ -\cos \Psi \cos \Phi & \cos \Psi \sin \Phi & -\sin \Psi \end{array} \right) \left( \begin{array}{l} \mathbf{F}_{1} \\ \mathbf{F}_{2} \\ \mathbf{F}_{3} \end{array} \right) . \end{equation*} |
Hence, the Blaschke equations are given as the following matrix:
\begin{equation*} \frac{d}{d\varphi }\left( \begin{array}{l} \mathbf{X} \\ \mathbf{T} \\ \mathbf{G} \end{array} \right) = \left( \begin{array}{lll} \ \ 0 &\ P & \ \ 0 \\ -P & \ \ 0 &\ Q \\ \ \ 0 & -Q & \ \ 0 \end{array} \right) \left( \begin{array}{l} \mathbf{X} \\ \mathbf{T} \\ \mathbf{G} \end{array} \right) , \end{equation*} |
where
\begin{equation} P = (1+\varepsilon h)\sin \Psi , \text{ }Q = (1+\varepsilon h)\cos \Psi \text{, and }\cot \Psi = \frac{Q}{P}. \end{equation} | (65) |
Thus we reach, by computing the real and dual parts of Eq (65), to the result
\begin{equation} \mu = h+\psi ^{\ast }\cot \psi \text{, }\Gamma = h-\psi ^{\ast }\tan \psi , \text{ }\gamma = \tan \psi , \end{equation} | (66) |
where \mu , \Gamma , and \gamma are constants. Hence, as a direct consequence of Definition 2, ( X ) is a constant parameter ruled surface. We can also show that < \frac{d\mathbf{r}}{d\varphi }, \frac{d\mathbf{x}}{ d\varphi } > = 0 ; so the base curve \mathbf{r}(\varphi) of ( X ) is its striction curve. Also, the curvature and the torsion of \mathbf{r}(\varphi) can be given, respectively, by
\begin{equation*} \kappa (\varphi ) = \frac{\psi ^{\ast }}{\psi ^{\ast 2}+h^{2}}\text{, and } \tau (\varphi ) = \frac{h}{\psi ^{\ast 2}+h^{2}}, \end{equation*} |
which leads to \mathbf{r}(\varphi) is a circular helix. Therefore, the constant parameter ruled surface can be characterized as follows:
(1) The constant helicoid: If we take h = 1 , \psi = \frac{\pi }{4} , \psi ^{\ast } = 1 , \ \varphi \in \lbrack -\pi, \pi] , and v\in \lbrack -4, 4] , the surface is shown in Figure 3. (2) The one-sheeted circular hyperboloid: Figure 4 shows the surface with \psi = \frac{\pi }{4} , \psi ^{\ast } = 1 , \varphi \in \lbrack -\pi, \pi] , v\in \lbrack -4, 4] , and h = 0 . (3) Archimedes helicoid: Figure 5 shows the surface with \psi = \frac{\pi }{4} , h = 1 , \ \varphi \in \lbrack -\pi, \pi] , v\in \lbrack -4, 4] , and \psi ^{\ast } = 0 . (4) A circular cone: Figure 6 shows the surface with \psi = \frac{ \pi }{4} , \ \varphi \in \lbrack -\pi, \pi] , v\in \lbrack -4, 4] , and \psi ^{\ast } = h = 0 .
Case (II). If \Sigma = 0 , then we have:
\begin{equation} \mathbf{X}^{^{^{\prime \prime \prime }}}+\mathbf{X}^{\prime } = \mathbf{0}. \end{equation} | (67) |
Also, from Eq (26), we can see that
\begin{equation} \Sigma = 0\Leftrightarrow \cot \Psi = 0\Leftrightarrow \psi = 0, \; \text{and} \; \psi ^{\ast } = 0. \end{equation} | (68) |
In such instant, the lines \mathbf{X} , \mathbf{T} and \mathbf{B} construct the Blaschke frame and meet at the striction point of the (X). Moreover, the oriented lines \mathbf{B} and \mathbf{G} coincide, That is, the line \mathbf{X} moves with respect to \mathbf{B} with constant pitch. Hence, we have a great circle on \mathbb{K}_{f} is described by
\begin{equation*} \mathbb{S} = \left \{ \mathbf{X}(S)\in \mathbb{K}_{f}\mid \mathbf{ \lt X}, \mathbf{B} \gt = 0\text{, with }\left \Vert \mathbf{B}\right \Vert ^{2} = 1\right \} . \end{equation*} |
Therefore, we conclude that all lines of (X) intersect perpendicularly the Disteli-axis \mathbf{ B} . Quite similarly as we did, we obtain
\begin{equation*} \mathbf{X}(\widehat{s}) = \left( -\cos \widehat{s}, \sin \widehat{s}, 0\right) . \end{equation*} |
The central normal can be found as \mathbf{T}(\widehat{s}) = (\sin \widehat{s}, \cos \widehat{s}, 0) . Thus \mathbf{G} has the form \mathbf{G} = (0, 0, -1) . Furthermore, an analogous arguments show that: \mu = h , and \gamma = \Gamma = 0 . Also, it can be shown that the ruled surface (X) is
\begin{equation} x = v\cos s, \ y = hs, \ \text{and }z = v\sin s. \end{equation} | (69) |
Or, in Cartesian coordinates,
\begin{equation} y = h\cot ^{-1}\left( \frac{x}{z}\right) , \end{equation} | (70) |
which represents a one-parameter family of right helicoids. If we take h = 1 , 0\leq s\leq 2\pi , and -3\leq v\leq 3 , then a member of such family can be formed, see Figure 7.
Corollary 2.. Let \mathbf{X} = \mathbf{X}(\widehat{s}) be a dual spherical curve. Then, the curve \mathbf{X} is a great circle if its dual geodesic curvature function \Sigma = \Sigma (\widehat{s}) vanishes. Moreover, the ruled surface is classified as a right helicoid and its striction curve is geodesic.
This paper presents a new approach for constructing ruled surfaces having constant Disteli-axis by means of the E. Study map. In terms of this, for the motion of the Blaschke frame on a regular dual spherical curve, some well-known formulae of surfaces theory into line space and their geometrical explanations are investigated. Finally, the geometric invariants of a line trajectory undrgoing one-parameter scrow motion are examined. The study of spatial kinematics in Euclidean 3-space \mathbb{E}^{3} via the E. Study map may used to solve some problems and conclude new applications.
The project was funded by the Deanship of Scientific Research (DSR), King Abdulaziz University, Jeddah, under grant no. D-191-662-1441. The authors, therefore, gratefully acknowledge the DSR technical and financial support.
Author is thankful to Prof. Rashad A. Abel-Baky for related discussions and to referees and reviewers who provided useful and detailed comments that helped to improve the manuscript.
Author has no conflict of interest.
[1] | R. A. Abdel-Baky, Inflection and torsion line Congruences, J. Geom. Graph., 11 (2007), 1-14. |
[2] | R. A. Abdel-Baky, On the curvature theory of a line trajectory in spatial kinematics, Commun. Korean Math. Soc., 34 (2019), 333-349. |
[3] | R. A. Abdel-Baky, R. A. Al-Ghefari, On the one-parameter dual spherical motions, Comput. Aided Geom. Design, 28 (2011), 23-37. |
[4] | R. A.Abdel-Baky, F. R. Al-Solamy, A new geometrical approach to one-parameter spatial motion, J. Eng. Math., 60 (2008), 149-172. |
[5] | R. A. Al-Ghefari, R. A. Abdel-Baky, Kinematic geometry of a line trajectory in spatial motion, J. Mech. Sci. Technol., 29 (2015), 3597-3608. |
[6] | O. Bottema, B. Roth, Theoretical Kinematics, North-Holland Press, New York, 1979. |
[7] | G. Figliolini, H. Stachel, J. Angeles, On Martin Disteli's spatial cycloidal gearing, Mech. Machine Theory, 60 (2012), 73-89. |
[8] | G. Figliolini, H. Stachel, J. Angeles, A new look at the Ball-Disteli diagram and its relevance to spatial gearing, Mech. Machine Theory, 42 (2007), 1362-1375. |
[9] | D. B. Dooner, M. W. Griffis, On spatial Euler-savary equations for envelopes, ASME J. Mech. Design, 129 (2006), 865-875. |
[10] | A. Karger, J. Novak, Kinematics and Lie Groups, Gordon and Breach Science Publishers, New York, 1985. |
[11] | H. Pottman, J. Wallner, Computational Line Geometry, Springer-Verlag, Berlin, Heidelberg, 2001. |
[12] | H. Stachel, Instantaneous Spatial Kinematics and the Invariants of the Axodes, Institute fur Geometrie, TU Wien, Technical Report 34, 1996. |
[13] | J. Tölke, Contributions to the theory of the axes of curvature, Mech. Machine Theory, 11 (1976), 123-130. |
[14] | D. Wang, J. Liu, D. Xiao, A unified curvature theory in kinematic geometry of mechanism, Sci. China (Series E), 41 (1998), 196-202. |
[15] | D. Wang, J. Liu, D. Xiao, Geometrical characteristics of some typical spatial constraints, Mech. Machine Theory, 35 (2000), 1413-1430. |
1. | Monia F. Naghi, Rashad A. Abdel-Baky, Fatemah Mofarreh, Gueo Grantcharov, Time-like Ruled Surface in One-Parameter Hyperbolic Dual Spherical Motions, 2022, 2022, 1687-0409, 1, 10.1155/2022/9323490 | |
2. | Nadia Alluhaibi, Rashad A. Abdel-Baky, Kinematic Geometry of Timelike Ruled Surfaces in Minkowski 3-Space E13, 2022, 14, 2073-8994, 749, 10.3390/sym14040749 | |
3. | Yanlin Li, Nadia Alluhaibi, Rashad A. Abdel-Baky, One-Parameter Lorentzian Dual Spherical Movements and Invariants of the Axodes, 2022, 14, 2073-8994, 1930, 10.3390/sym14091930 | |
4. | Nadia Alluhaibi, Rashad A. Abdel-Baky, Kinematic-geometry of lines with special trajectories in spatial kinematics, 2023, 8, 2473-6988, 10887, 10.3934/math.2023552 | |
5. | Areej A. Almoneef, Rashad A. Abdel-Baky, On the axodes of one-parameter spatial movements, 2024, 9, 2473-6988, 9867, 10.3934/math.2024483 | |
6. | Awatif Al-Jedani, Rashad A. Abdel-Baky, One-parameter Lorentzian spatial kinematics and Disteli's formulae, 2023, 8, 2473-6988, 20187, 10.3934/math.20231029 | |
7. | Yanlin Li, Fatemah Mofarreh, Rashad A. Abdel-Baky, Kinematic-geometry of a line trajectory and the invariants of the axodes, 2023, 56, 2391-4661, 10.1515/dema-2022-0252 | |
8. | Areej A. Almoneef, Rashad A. Abdel-Baky, Kinematic Differential Geometry of a Line Trajectory in Spatial Movement, 2023, 12, 2075-1680, 472, 10.3390/axioms12050472 | |
9. | Areej A. Almoneef, Rashad A. Abdel-Baky, Spacelike Lines with Special Trajectories and Invariant Axodes, 2023, 15, 2073-8994, 1087, 10.3390/sym15051087 | |
10. | Areej A. Almoneef, Rashad A. Abdel-Baky, One-Parameter Hyperbolic Spatial Locomotions and Invariants of the Axode, 2023, 11, 2227-7390, 3749, 10.3390/math11173749 | |
11. | Awatif Al-Jedani, On the Curvature Functions of Ruled Surface, 2024, 34, 0218-1959, 25, 10.1142/S021819592450002X |