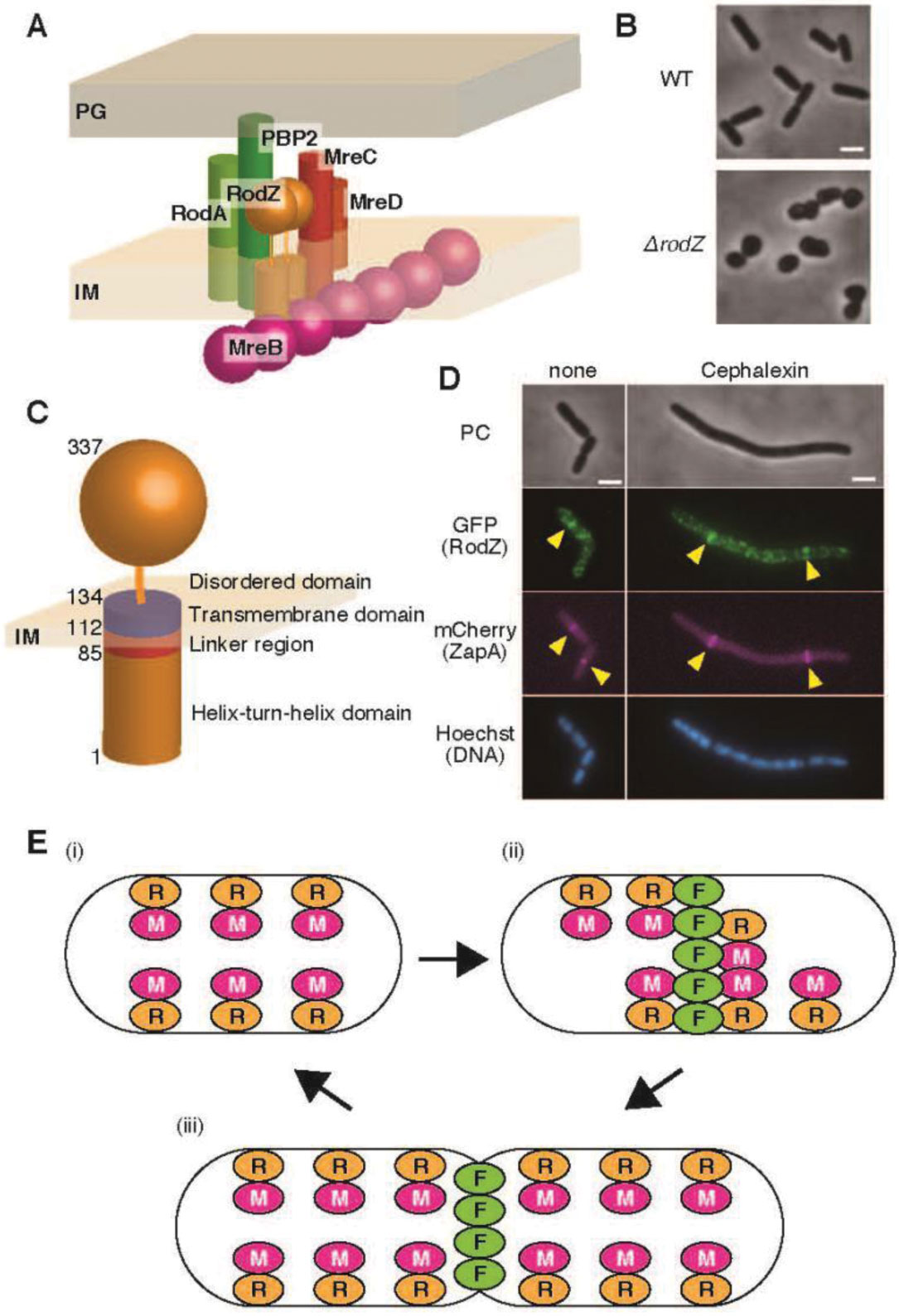
Citation: Risa Ago, Daisuke Shiomi. RodZ: a key-player in cell elongation and cell division in Escherichia coli[J]. AIMS Microbiology, 2019, 5(4): 358-367. doi: 10.3934/microbiol.2019.4.358
[1] | Yusuke Morita, Mai Okumura, Issay Narumi, Hiromi Nishida . Sensitivity of Deinococcus grandis rodZ deletion mutant to calcium ions results in enhanced spheroplast size. AIMS Microbiology, 2019, 5(2): 176-185. doi: 10.3934/microbiol.2019.2.176 |
[2] | Chiaki Sugiura, Saki Miyaue, Yuka Shibata, Akiko Matsumoto, Sumio Maeda . Bacteriophage P1vir-induced cell-to-cell plasmid transformation in Escherichia coli. AIMS Microbiology, 2017, 3(4): 784-797. doi: 10.3934/microbiol.2017.4.784 |
[3] | Elena Kotenkova, Dagmara Bataeva, Mikhail Minaev, Elena Zaiko . Application of EvaGreen for the assessment of Listeria monocytogenes АТСС 13932 cell viability using flow cytometry. AIMS Microbiology, 2019, 5(1): 39-47. doi: 10.3934/microbiol.2019.1.39 |
[4] | Luciana C. Gomes, Filipe J. Mergulhão . Effect of heterologous protein expression on Escherichia coli biofilm formation and biocide susceptibility. AIMS Microbiology, 2016, 2(4): 434-446. doi: 10.3934/microbiol.2016.4.434 |
[5] | Afraa Said Al-Adawi, Christine C. Gaylarde, Jan Sunner, Iwona B. Beech . Transfer of bacteria between stainless steel and chicken meat: A CLSM and DGGE study of biofilms. AIMS Microbiology, 2016, 2(3): 340-358. doi: 10.3934/microbiol.2016.3.340 |
[6] | Anil K. Persad, Michele L. Williams, Jeffrey T. LeJeune . Rapid loss of a green fluorescent plasmid in Escherichia coli O157:H7. AIMS Microbiology, 2017, 3(4): 872-884. doi: 10.3934/microbiol.2017.4.872 |
[7] | Yuichi Hakumai, Kanae Yamaguchi, Sawami Nakayama, Makoto Ashiuchi . Effective elimination of water-borne Escherichia coli using archaeal poly-g-glutamate-based materials. AIMS Microbiology, 2016, 2(3): 222-229. doi: 10.3934/microbiol.2016.3.222 |
[8] | Luciana C. Gomes, Joana M. R. Moreira, José D. P. Araújo, Filipe J. Mergulhão . Surface conditioning with Escherichia coli cell wall components can reduce biofilm formation by decreasing initial adhesion. AIMS Microbiology, 2017, 3(3): 613-628. doi: 10.3934/microbiol.2017.3.613 |
[9] | Joseph O. Falkinham . Mycobacterium avium complex: Adherence as a way of life. AIMS Microbiology, 2018, 4(3): 428-438. doi: 10.3934/microbiol.2018.3.428 |
[10] | Natalia Y. Kovalskaya, Eleanor E. Herndon, Juli A. Foster-Frey, David M. Donovan, Rosemarie W. Hammond . Antimicrobial activity of bacteriophage derived triple fusion protein against Staphylococcus aureus. AIMS Microbiology, 2019, 5(2): 158-175. doi: 10.3934/microbiol.2019.2.158 |
Bacterial cells repeatedly elongate and divide to proliferate, but these two events must be precisely coordinated. Cell elongation is regulated by a supramolecular complex called Rod complex (Figure 1A), while cell division is regulated by a supramolecular complex called divisome. MreB—prokaryotic homolog of actin, and FtsZ—prokaryotic homolog of tubulin are scaffold proteins for the Rod complex and the divisome, respectively [1]. Rod complex consists of many proteins, such as MreB, a transmembrane protein RodZ, and a peptidoglycan synthesis enzyme penicillin-binding protein (PBP) 2; divisome consists of many proteins, such as FtsZ, ZipA, ZapA, and a peptidoglycan synthesis enzyme PBP3 [1],[2]. The shape of a bacterial cell is determined by a balance between elongation and division. These two events were initially thought to be independent; however, it was found that MreB localizes to the division site in an FtsZ-dependent manner [3]–[5] and PBP2 directly interacts with PBP3 [6], suggesting that the Rod complex interacts with the divisome at the division site. Furthermore, it was shown that the direct interaction between MreB and FtsZ is important for the transition from cell elongation to cell division [7]. We recently found that the transmembrane protein RodZ also localizes to the divisome and facilitates the formation of the divisome [8]. This mini-review outlines the features of RodZ, especially focusing on our recent results. Here, we describe RodZ of Escherichia coli unless otherwise stated.
rodZ gene was identified independently by three research groups more than ten years ago [9]–[11]. We visually screened the KEIO knockout library (3985 strains), which is a collection of single-gene knockouts of non-essential genes in E. coli, to find mutant(s) that showed abnormal morphology. One of the mutants in the library (ΔyfgA) showed round or ovoid-shaped phenotype (Figure 1B) [9]. de Boer's group also searched for a transposon insertion mutant that required additional FtsZ for viability or better growth and showed cell-shape defects like other cell-shape mutants, such as the mreB mutant [12]. Consequently, they found a mutant in which transposon was inserted into the yfgA gene. yfgA was then renamed rodZ because the mutant required an overdose of FtsZ for propagation in LB [10]. Furthermore, Jacobs-Wagner's group also visually screened a transposon library of Caulobacter crescentus and found a mutant which showed slow growth and aberrant morphology [11]. In the mutant, a transposon was inserted into cc_0850, which is a homolog of rodZ, indicating that RodZ plays a role in morphogenesis in C. crescentus (like in E. coli). It has also been shown that RodZ is required for cell shape maintenance in Bacillus subtilis and Deinococcus grandis [13],[14]. All the above reports showed that rodZ mutants exhibit aberrant morphology, indicating that RodZ is required for proper cell-shape maintenance. It should be noted that rodZ is a non-essential gene, whereas most of the other cell shape determinants, such as mreB act as essential genes in rich medium [12].
RodZ is a bitopic membrane protein of 337 amino acid residues (Figure 1C). It has a helix-turn-helix (HTH) domain (RodZ1−84) in the N-terminal cytoplasmic domain (amino acid residues 1–111). [15]. Although this domain belongs to the XRE family of transcription factors, the HTH domain does not interact with DNA, but it interacts with MreB and RodZ itself [10],[15]. Consistent with these results, it has also been reported that RodZ is not involved in segregation of chromosome in E. coli [10]. The HTH domain is followed by a linker region (RodZ85−111), also called the juxta-membrane region, which contains many positively charged residues that are not important for the key functions of RodZ, such as cell shape maintenance [16]. The functions of the transmembrane domain of RodZ (RodZ112−133) are currently under investigation in our lab (Ago et al., unpublished). The periplasmic domain of RodZ is largely dispensable because cells producing RodZ1−142 or RodZ1−155, which lacks most of the region, show a rod shape [9]. The structure of the periplasmic domain of RodZ from B. subtilis was predicted by NMR [17]. According to the prediction, the periplasmic domain is composed of disordered domains and is followed by a folded domain that contains nine ß-sheets. We showed that RodZ forms at least one dimer through its disordered domain, by in vivo site-specific photo-cross-linking assay [18]. Therefore, RodZ self-interacts in the cytoplasm and in the periplasm.
As described above, rodZ mutants of E. coli, C. crescentus, B. subtilis, and D. grandis showed aberrant morphology, such as round, ovoid, or shorter-rod phenotypes [9]–[11],[13],[14]. On the other hand, overproduction of RodZ resulted in elongation [9] or swelling [10] of the bacterial cells. This apparent contradiction may be explained by the amount of RodZ and MreB present in the cells, in other words, by the ratio of molecular number of RodZ and MreB. Because simultaneous overproduction of RodZ and MreB also resulted in cell elongation [10], ‘slight’ overproduction of RodZ may help MreB to assemble so that cells are able to elongate. On the other hand, ‘enormous’ overproduction of RodZ may result in perturbation of the Rod complex, causing cell elongation failure. All these results indicate that RodZ is an important factor in the maintenance of proper bacterial cell-shape. MreB is generally conserved in rod-shaped bacteria [19]. However, interestingly, RodZ is widely conserved among bacterial species, including several cocci that even lack mreB (such as Streptococcus spp. and Staphylococcus spp.) [11],[20]. This suggests that RodZ has a general role in cell morphogenesis, such as regulation of peptidoglycan synthesis. Consistent with this hypothesis, it was observed that RodZ in C. crescentus localizes at sites where peptidoglycan is synthesized [11].
Subcellular localization of RodZ has been previously examined. We originally observed the localization of green fluorescent protein (GFP)-RodZ expressed from a plasmid [9] and de Boer's lab observed GFP-RodZ expressed from chromosomal attHK022 site [10]. Recently, we constructed strains expressing super folder GFP (sfGFP)-RodZ or monomeric sfGFP (msfGFP) -RodZ from its native locus [8],[18]. It should be noted that RodZ fused with GFP (or its variants) is fully capable of maintaining the rod shape. These experiments showed that RodZ formed clusters or spiral-like structures in the cylindrical part of a cell (Figure 1D). This localization pattern of RodZ was reminiscent of that of MreB [21],[22], a major component of the Rod complex, suggesting that RodZ is also involved in the Rod complex. In the absence of MreB, RodZ was distributed throughout the cytoplasmic membrane, indicating that MreB directs localization of RodZ [10]. However, when cells producing GFP-RodZ were treated with A22, a drug that inhibits assembly of MreB [23],[24], GFP-RodZ still showed clusters or filament-like pattern. This suggests that MreB is not required for maintenance of RodZ clusters, but is required for their initial assembly. MreB-mCherrySW, in which mCherry was inserted between G228 and D229 of MreB, was expressed from its native locus and formed aberrant clusters in ΔrodZ cells [10]. On the other hand, GFP-MreB, which was expressed from a plasmid, still showed filamentous structures in ΔrodZ cells [9]. This suggests that RodZ is required for proper assembly of MreB, although MreB, when overproduced, can form clusters or filaments in the absence of RodZ. It should be noted that GFP-MreB fusion is not functional and that it was reported that the helical structures observed using YFP-MreB were artifacts in E. coli [25]. Therefore, the results from experiments using GFP-MreB fusion are hard to interpret. It was shown that RodZ does not affect polymer length of MreB (WT = 515 ± 15 nm, ΔrodZ = 509 ± 18 nm), but significantly affects total MreB polymer number per cell (WT = 11.6 ± 0.5, ΔrodZ = 6.6 ± 0.3) [26]. Bimolecular fluorescence complementation (BiFC) has been used to show that MreB interacts with RodZ, and that RodZ1−142 and RodZ1−111 still interact with MreB [27], supporting the above results. Interestingly, MreB polymer numbers per cell in cells producing RodZ1−142 or RodZ1−111 were decreased compared with those in WT cells [26]. These results suggest that RodZ regulates MreB polymer number directly and indirectly through other proteins.
Biochemical analyses revealed that RodZ directly interacts with MreB in vitro [15]. Purified RodZ1−104, the cytoplasmic fragment of RodZ from Thermotoga maritima, interacts with both monomeric and oligomeric MreB from T. maritima. The structure of RodZ1−88 from T. maritima was solved as a co-crystal with MreB [15]. The authors mutated the binding sites in RodZ molecule and concluded that RodZ interacts with subdomain IIA in MreB. T. maritima RodZY53A and RodZY57A (corresponding to E. coli RodZF60A and RodZY64A, respectively) mutants, in which mutation sites are located at the interface with subdomain IIA of MreB, decreased RodZ binding ability to MreB. In addition to BiFC, fluorescence resonance energy transfer (FRET) and bacterial two-hybrid (BACTH) assays also revealed that RodZ interacts with MreB in vivo [15],[28]. Furthermore, it was observed that GFP-RodZ1−138−F60A-RFP and sfGFP-RodZF60A did not form clusters, but were diffused [8],[15], consistent with the result that RodZF60A had decreased interaction with MreB. This suggests that MreB is responsible for localization of RodZ. It has been observed that MreB localizes to the cylindrical part of a rod-shaped cell. This localization pattern is accomplished by two factors; curvature-dependent cylindrical localization of MreB [29] and exclusion of MreB from cell poles by anionic phospholipids [30]. RodZ regulates curvature sensing and polymer numbers of MreB, although it is still controversial [26],[31],[32]. Thus, it can be said that RodZ might regulate localization of MreB. MreB is not static but highly dynamic; MreB rotates perpendicular to the long axis of a cell [33]–[35], and this rotation is not absolutely required for a rod shape because ΔrodZ cells producing MreBS14A, which does not rotate, can form a rod shape, although they are slightly fat [27]. RodZ shows the same motion as MreB and plays a role in linking between MreB and PBP2/RodA, which are peptidoglycan synthases [27]. In summary, RodZ and MreB are important to each other's clustering and localization and motion of the Rod complex.
Besides MreB, RodZ interacts with multiple proteins involved in the Rod complex. RodZ interacts with MreC, MreD, PBP2, and RodA, as shown in BACTH and Bimolecular fluorescence complementation (BiFC) assays [10],[27]. We also revealed genetic interaction between RodZ and MreB, RodA and PBP2 [36]. Growth of cells lacking rodZ is slower than that of WT cells. We isolated suppressor mutants that restored the growth defect of ΔrodZ cells [36]. These suppressors also restored rod shape, suggesting a connection between cell shape and growth rate in E. coli. Faster-growing rodZ mutants are also suppressed for the shape defect, as most of the mutants grew more rod-like than did ΔrodZ cells. This indicates a connection between cell shape and growth rate in E. coli. Whole genome sequencing of the suppressor mutants identified the mutation sites and showed that twenty suppressors had a mutation in the mreB gene (e.g., mreBA125V), four suppressors had a mutation in mrdA (encoding PBP2), two suppressors had a mutation in mrdB (encoding RodA), and one suppressor had a mutation in the promoter region of zipA. These proteins, except for ZipA, are components of the Rod complex. Therefore, this strongly suggests that RodZ is also a component of the Rod complex. The mutation sites on the three-dimensional structure of MreB were mostly mapped at the lateral contact sites of the antiparallel double filaments of MreB [36],[37]. This suggests that RodZ modulates the lateral contact of MreB filaments. We also isolated suppressor mutants of a chimeric protein in which the transmembrane domain of RodZ was replaced by another protein and found mutations in mreC, mreD, mrdA, mrdB in addition to mreB (Ago et al., manuscript in preparation). These suppressor mutations were mapped on protein-protein interaction surfaces, suggesting that RodZ modulates protein-protein interaction in the Rod complex in addition to the regulation of subcellular localization of MreB.
During proliferation, cells elongate and divide continuously. When cells divide, they change the sites of peptidoglycan synthesis from the cylindrical part to the division site. PBP2, an elongation-specific peptidoglycan synthesis enzyme, and PBP3, a division-specific peptidoglycan synthesis enzyme, colocalize during the early period of maturation of the division machinery [6], indicating that the Rod complex and the divisome interact with each other for transition from cell elongation to division. It was also shown that MreB localizes to the division site in an FtsZ-dependent manner [4],[5] and MreB directly interacts with FtsZ [7]. The interaction between MreB and FtsZ is required for the transition from elongation to division because cells producing MreBD285A, which does not interact with FtsZ, do not divide but can elongate [7]. Because RodZ directly interacts with MreB [15] and possibly modulates a property of MreB filaments [36], it is possible that RodZ also localizes to the division site. We recently found that RodZ also localized to the division site in an FtsZ-dependent manner (Figure 1D) [8]. RodZ directly interacts with FtsZ through the N-terminal cytoplasmic domain. Additionally, localization of ZapA, a component of the divisome, was delayed when RodZ did not localize to the division site. These results suggest that RodZ is not essential for cell division, but accelerates or stabilizes the divisome for efficient division. It is plausible that MreB recruits RodZ to the division site. In fact, RodZ does not localize to the division site in cells lacking mreB. However, unexpectedly, we also found that RodZF60A, which decreases the binding ability of RodZ to MreB [15], localized to the division site. Only 4.7% of the cells in the population showed division site localization of MreB in cells producing RodZF60A. Furthermore, overproduction of RodZF60A increased the proportion of cells showing localization of MreB (27.3%) to the division site. These results suggest that RodZ is required for the division site localization of MreB. Consistent with these results, MreBA125V did not localize to the division site in ΔrodZ cells. It should be noted that ΔrodZ cells producing MreBA125V are rod-shaped because the A125V mutation of MreB serves as a suppressor mutation of ΔrodZ.
As described above, cells cannot divide but can elongate if MreB does not localize to the division site [7]. However, cells producing RodZF60A are able to divide although, MreB does not localize to the division site, suggesting that the division site localization of MreB is not required for cell division [8]. We can explain this apparent contradiction as follows. Proliferation of bacterial cells is determined by a balance between peptidoglycan synthesis for elongation by the Rod complex and for division by divisome. MreBD285A does not interact with FtsZ, but with RodZ and MreC, such that the MreB mutant is able to form the Rod complex. Thus, cells producing MreBD285A continue to elongate. On the other hand, RodZ does not localize to the division site in ΔmreB cells. Interestingly, RodZF60A localizes to the division site and cells producing RodZF60A are able to elongate and divide. RodZF60A can interact with FtsZ and MreB although the interaction between RodZF60A and MreB is significantly weaker than that between WT RodZ and MreB [8]. This results in formation of a ‘weak’ Rod complex. Interaction between RodZ and MreB may be required for conformational change of RodZ to a suitable structure for division site localization. Weak interaction between MreB and RodZF60A may be sufficient for the structural change of RodZ. Therefore, the weak Rod complex can localize to the division site and promote cell division. The balance between peptidoglycan synthesis for elongation by the Rod complex and for division by the divisome may be appropriate in cells producing RodZF60A. Thus, cells producing RodZF60A are able to divide, although MreB does not localize properly to the division site in the same cell. In the absence of MreB, the structure of RodZ fails to change to the suitable structure for division site localization, so that RodZ does not localize to the division site. Another interesting result in our study is that RodZ85−337 and RodZ104−337, which lack most of the cytoplasmic domain and clearly have a decreased ability to interact with FtsZ, still localized to the division site, suggesting that the periplasmic domain of RodZ interacts with the periplasmic domain of a component(s) of the divisome; PBP3 (also called FtsI) could be this component because it is a division-specific peptidoglycan synthesis enzyme and interacts with PBP2. This interaction would also be important for transition from cell elongation to division or stabilization of the divisome for cell division. Taken together, RodZ plays roles in both cell elongation and cell division (Figure 1F).
Several reports have revealed interactions among proteins of the Rod complex and the divisome, and the biological significance of those interactions. In E. coli, the Rod complex and the divisome colocalize at the division site during the early stages of cell division [6]. It has been shown that MreB localizes to the division site in an FtsZ-dependent manner [4],[22] and it directly interacts with FtsZ [7]. RodZ also localizes to the division site, but the division site localization of RodZ is not essential for cell division because even ΔrodZ cells can divide; however, it has been shown that RodZ promotes the formation of the divisome during the early stages of cell division [8]. In C. crescentus also, RodZ localizes to the division site. This localization is dependent on both FtsZ and MreB, which means that MreB might be recruiting RodZ to the division site [11]. The authors showed that GFP-RodZ did not localize to the division site in cells producing MreBQ26P, which is a mutant that is unable to localize to the division site. However, cells producing MreBQ26P had normal shape and divided properly, suggesting that RodZ and MreB are not critical for cell division in C. crescentus. In C. crescentus and E. coli, FtsZ directs peptidoglycan synthesis for elongation, which is called PIPS (PBP3-independent peptidoglycan synthesis) or preseptal elongation, at the division site. RodZ may be involved in PIPS. RodZ is widely conserved among various bacterial cells, including ovoid-shaped bacteria that lack mreB, such as Streptococcus pneumoniae and Staphylococcus aureus. In ovoid-shaped S. pneumoniae cells, there are two types of peptidoglycan synthesis; peripheral growth and septal growth [38]. Peripheral growth in S. pneumoniae may be similar to PIPS in E. coli. Thus, RodZ in S. pneumoniae may play a role in the peripheral growth because MreC and MreD, whose homologs are components of the Rod complex in E. coli, are required for peripheral growth [39]. On the other hand, in S. aureus, MreC and MreD localize to the division site, but are not critical for cell division and cell shape determination [40]. Therefore, RodZ may not be involved in the regulation of cell division in S. aureus. In Chlamydia, which has no homolog of FtsZ: an important protein for cell division in the majority of bacterial species, MreB plays a role in cell division [41] and RodZ interacts with MreB [42],[43]. Thus, RodZ in Chlamydia may also be important for cell division, although the underlying molecular mechanism is still unknown. All these reports suggest that biological functions of the division site localization of RodZ vary in different bacterial species. There are several reports on the roles of RodZ in other cellular processes such as regulation of post-transcription of type III secretion, biofilm formation, and spatial regulation of other proteins [16],[44]–[47]; these should be discussed in the future to understand bona fide roles of RodZ in different cellular processes, including cell division and cell elongation.
[1] |
Szwedziak P, Löwe J (2013) Do the divisome and elongasome share a common evolutionary past? Curr Opin Microbiol 16: 745–751. doi: 10.1016/j.mib.2013.09.003
![]() |
[2] |
Blaauwen den T, de Pedro MA, Nguyen-Distèche M, et al. (2008) Morphogenesis of rod-shaped sacculi. FEMS Microbiol Rev 32: 321–344. doi: 10.1111/j.1574-6976.2007.00090.x
![]() |
[3] |
Figge RM, Divakaruni AV, Gober JW (2004) MreB, the cell shape-determining bacterial actin homologue, co-ordinates cell wall morphogenesis in Caulobacter crescentus. Mol Microbiol 51: 1321–1332. doi: 10.1111/j.1365-2958.2003.03936.x
![]() |
[4] |
Vats P, Rothfield L (2007) Duplication and segregation of the actin (MreB) cytoskeleton during the prokaryotic cell cycle. Proc Natl Acad Sci USA 104: 17795–17800. doi: 10.1073/pnas.0708739104
![]() |
[5] |
Vats P, Shih YL, Rothfield L (2009) Assembly of the MreB-associated cytoskeletal ring of Escherichia coli. Mol Microbiol 72: 170–182. doi: 10.1111/j.1365-2958.2009.06632.x
![]() |
[6] |
van der Ploeg R, Verheul J, Vischer NOE, et al. (2013) Colocalization and interaction between elongasome and divisome during a preparative cell division phase in Escherichia coli. Mol Microbiol 87: 1074–1087. doi: 10.1111/mmi.12150
![]() |
[7] |
Fenton AK, Gerdes K (2013) Direct interaction of FtsZ and MreB is required for septum synthesis and cell division in Escherichia coli. EMBO J 32: 1953–1965. doi: 10.1038/emboj.2013.129
![]() |
[8] |
Yoshii Y, Niki H, Shiomi D (2019) Division-site localization of RodZ is required for efficient Z ring formation in Escherichia coli. Mol Microbiol 111: 1229–1244. doi: 10.1111/mmi.14217
![]() |
[9] |
Shiomi D, Sakai M, Niki H (2008) Determination of bacterial rod shape by a novel cytoskeletal membrane protein. EMBO J 27: 3081–3091. doi: 10.1038/emboj.2008.234
![]() |
[10] |
Bendezú FO, Hale CA, Bernhardt TG, et al. (2009) RodZ (YfgA) is required for proper assembly of the MreB actin cytoskeleton and cell shape in E. coli. EMBO J 28: 193–204. doi: 10.1038/emboj.2008.264
![]() |
[11] |
Alyahya SA, Alexander R, Costa T, et al. (2009) RodZ, a component of the bacterial core morphogenic apparatus. Proc Natl Acad Sci USa 106: 1239–1244. doi: 10.1073/pnas.0810794106
![]() |
[12] |
Bendezú FO, de Boer PAJ (2008) Conditional lethality, division defects, membrane involution, and endocytosis in mre and mrd shape mutants of Escherichia coli. J Bacteriol 190: 1792–811. doi: 10.1128/JB.01322-07
![]() |
[13] |
Muchová K, Chromiková Z, Barák I (2013) Control of Bacillus subtilis cell shape by RodZ. Environ Microbiol 15: 3259–3271. doi: 10.1111/1462-2920.12200
![]() |
[14] |
Okumura M, Narumi I, Nishida H (2019) Sensitivity of Deinococcus grandis rodZ deletion mutant to calcium ions results in enhanced spheroplast size. AIMS Microbiology 5: 176–185. doi: 10.3934/microbiol.2019.2.176
![]() |
[15] |
van den Ent F, Johnson CM, Persons L, et al. (2010) Bacterial actin MreB assembles in complex with cell shape protein RodZ. EMBO J 29: 1081–1090. doi: 10.1038/emboj.2010.9
![]() |
[16] |
Mitobe J, Yanagihara I, Ohnishi K, et al. (2011) RodZ regulates the post-transcriptional processing of the Shigella sonnei type III secretion system. EMBO Rep 12: 911–916. doi: 10.1038/embor.2011.132
![]() |
[17] |
Pereira AC, Paiva A, Saraiva IH, et al. (2015) Chemical shift assignments and secondary structure determination of the ectodomain of Bacillus subtilis morphogenic protein RodZ. Biomol NMR Assign 9: 285–288. doi: 10.1007/s12104-014-9593-8
![]() |
[18] |
Ikebe R, Kuwabara Y, Chikada T, et al. (2018) The periplasmic disordered domain of RodZ promotes its self-interaction in Escherichia coli. Genes Cells 23: 307–317. doi: 10.1111/gtc.12572
![]() |
[19] |
Daniel RA, Errington J (2003) Control of cell morphogenesis in bacteria: two distinct ways to make a rod-shaped cell. Cell 113: 767–776. doi: 10.1016/S0092-8674(03)00421-5
![]() |
[20] |
Philippe J, Vernet T, Zapun A (2014) The elongation of ovococci. Microb Drug Resist 20: 215–221. doi: 10.1089/mdr.2014.0032
![]() |
[21] |
Jones LJ, Carballido-López R, Errington J (2001) Control of cell shape in bacteria: helical, actin-like filaments in Bacillus subtilis. Cell 104: 913–922. doi: 10.1016/S0092-8674(01)00287-2
![]() |
[22] |
Shih YL, Le T, Rothfield L (2003) Division site selection in Escherichia coli involves dynamic redistribution of Min proteins within coiled structures that extend between the two cell poles. Proc Natl Acad Sci USA 100: 7865–7870. doi: 10.1073/pnas.1232225100
![]() |
[23] |
Bean GJ, Flickinger ST, Westler WM, et al. (2009) A22 disrupts the bacterial actin cytoskeleton by directly binding and inducing a low-affinity state in MreB. Biochemistry 48: 4852–4857. doi: 10.1021/bi900014d
![]() |
[24] |
Iwai N, Nagai K, Wachi M (2002) Novel S-benzylisothiourea compound that induces spherical cells in Escherichia coli probably by acting on a rod-shape-determining protein(s) other than penicillin-binding protein 2. Biosci Biotechnol Biochem 66: 2658–2662. doi: 10.1271/bbb.66.2658
![]() |
[25] |
Swulius MT, Jensen GJ (2012) The helical MreB cytoskeleton in Escherichia coli MC1000/pLE7 is an artifact of the N-Terminal yellow fluorescent protein tag. J Bacteriol 194: 6382–6386. doi: 10.1128/JB.00505-12
![]() |
[26] | Bratton BP, Shaevitz JW, Gitai Z, et al. (2018) MreB polymers and curvature localization are enhanced by RodZ and predict E. coli's cylindrical uniformity. Nat Commun 9: 2797. |
[27] |
Morgenstein RM, Bratton BP, Nguyen JP, et al. (2015) RodZ links MreB to cell wall synthesis to mediate MreB rotation and robust morphogenesis. Proc Natl Acad Sci USA 112: 12510–12515. doi: 10.1073/pnas.1509610112
![]() |
[28] |
van der Ploeg R, Goudelis ST, Blaauwen den T (2015) Validation of FRET assay for the screening of growth inhibitors of Escherichia coli reveals elongasome assembly dynamics. Int J Mol Sci 16: 17637–17654. doi: 10.3390/ijms160817637
![]() |
[29] |
Ursell TS, Nguyen J, Monds RD, et al. (2014) Rod-like bacterial shape is maintained by feedback between cell curvature and cytoskeletal localization. Proc Natl Acad Sci USA 111: E1025–E1034. doi: 10.1073/pnas.1317174111
![]() |
[30] |
Kawazura T, Matsumoto K, Kojima K, et al. (2017) Exclusion of assembled MreB by anionic phospholipids at cell poles confers cell polarity for bidirectional growth. Mol Microbiol 104: 472–486. doi: 10.1111/mmi.13639
![]() |
[31] |
Colavin A, Shi H, Huang KC (2018) RodZ modulates geometric localization of the bacterial actin MreB to regulate cell shape. Nat Commun 9: 1280. doi: 10.1038/s41467-018-03633-x
![]() |
[32] | Dion MF, Kapoor M, Sun Y, et al. (2019) Bacillus subtilis cell diameter is determined by the opposing actions of two distinct cell wall synthetic systems. Nat Microbiol 24: 96. |
[33] | Garner EC, Bernard R, Wang W, et al. (2011) Coupled, circumferential motions of the cell wall synthesis machinery and MreB filaments in B. subtilis. Science 333: 222–225. |
[34] |
Domínguez-Escobar J, Chastanet A, Crevenna AH, et al. (2011) Processive movement of MreB-associated cell wall biosynthetic complexes in bacteria. Science 333: 225–228. doi: 10.1126/science.1203466
![]() |
[35] |
van Teeffelen S, Wang S, Furchtgott L, et al. (2011) The bacterial actin MreB rotates, and rotation depends on cell-wall assembly. Proc Natl Acad Sci USA 108: 15822–15827. doi: 10.1073/pnas.1108999108
![]() |
[36] |
Shiomi D, Toyoda A, Aizu T, et al. (2013) Mutations in cell elongation genes mreB, mrdA and mrdB suppress the shape defect of RodZ-deficient cells. Mol Microbiol 87: 1029–1044. doi: 10.1111/mmi.12148
![]() |
[37] | van den Ent F, Izoré T, Bharat TA, et al. (2014) Bacterial actin MreB forms antiparallel double filaments. Elife 2014: e02634. |
[38] |
Pinho MG, Kjos M, Veening JW (2013) How to get (a)round: mechanisms controlling growth and division of coccoid bacteria. Nat Rev Microbiol 11: 601–614. doi: 10.1038/nrmicro3088
![]() |
[39] |
Land AD, Winkler ME (2011) The requirement for pneumococcal MreC and MreD is relieved by inactivation of the gene encoding PBP1a. J Bacteriol 193: 4166–4179. doi: 10.1128/JB.05245-11
![]() |
[40] |
Tavares AC, Fernandes PB, Carballido-Lopez R, et al. (2015) MreC and MreD Proteins are not required for growth of Staphylococcus aureus. PLoS One 10: e0140523. doi: 10.1371/journal.pone.0140523
![]() |
[41] |
Ouellette SP, Karimova G, Subtil A, et al. (2012) Chlamydia co-opts the rod shape-determining proteins MreB and Pbp2 for cell division. Mol Microbiol 85: 164–178. doi: 10.1111/j.1365-2958.2012.08100.x
![]() |
[42] | Ouellette SP, Rueden KJ, Gauliard E, et al. (2014) Analysis of MreB interactors in Chlamydia reveals a RodZ homolog but fails to detect an interaction with MraY. Front Microbiol 5: 279. |
[43] |
Kemege KE, Hickey JM, Barta ML, et al. (2015) Chlamydia trachomatis protein CT009 is a structural and functional homolog to the key morphogenesis component RodZ and interacts with division septal plane localized MreB. Mol Microbiol 95: 365–382. doi: 10.1111/mmi.12855
![]() |
[44] | Niba ETE, Li G, Aoki K, et al. (2010) Characterization of rodZ mutants: RodZ is not absolutely required for the cell shape and motility. FEMS Microbiol Lett 309: 35–42. |
[45] | Niba ETE, Naka Y, Nagase M, et al. (2007) A genome-wide approach to identify the genes involved in biofilm formation in E. coli. DNA Res 14: 237–246. |
[46] |
Jovanovic G, Mehta P, Ying L, et al. (2014) Anionic lipids and the cytoskeletal proteins MreB and RodZ define the spatio-temporal distribution and function of membrane stress controller PspA in Escherichia coli. Microbiology 160: 2374–2386. doi: 10.1099/mic.0.078527-0
![]() |
[47] | van Beilen J, Blohmke CJ, Folkerts H, et al. (2016) RodZ and PgsA play intertwined roles in membrane homeostasis of Bacillus subtilis and resistance to weak organic acid stress. Front Microbiol 7: 1015–1012. |
1. | Atsushi Yahashiri, Jill T. Babor, Ariel L. Anwar, Ryan P. Bezy, Evan W. Piette, S. J. Ryan Arends, Ute Müh, Monica R. Steffen, Jeremy M. Cline, David N. Stanek, Steven D. Lister, Shauna M. Swanson, David S. Weiss, Yves V. Brun, DrpB (YedR) Is a Nonessential Cell Division Protein in Escherichia coli, 2020, 202, 0021-9193, 10.1128/JB.00284-20 | |
2. | Hiromi Nishida, Factors That Affect the Enlargement of Bacterial Protoplasts and Spheroplasts, 2020, 21, 1422-0067, 7131, 10.3390/ijms21197131 | |
3. | Melissa M. Lamanna, Irfan Manzoor, Merrin Joseph, Ziyun A. Ye, Mattia Benedet, Alessia Zanardi, Zhongqing Ren, Xindan Wang, Orietta Massidda, Ho‐Ching T. Tsui, Malcolm E. Winkler, Roles of RodZ and class A PBP1b in the assembly and regulation of the peripheral peptidoglycan elongasome in ovoid‐shaped cells of Streptococcus pneumoniae D39 , 2022, 118, 0950-382X, 336, 10.1111/mmi.14969 | |
4. | Jia Xin Yee, Juhyun Kim, Jinki Yeom, Membrane Proteins as a Regulator for Antibiotic Persistence in Gram-Negative Bacteria, 2023, 1225-8873, 10.1007/s12275-023-00024-w | |
5. | Amilcar J. Perez, Melissa M. Lamanna, Kevin E. Bruce, Marc A. Touraev, Julia E. Page, Sidney L. Shaw, Ho-Ching Tiffany Tsui, Malcolm E. Winkler, Elongasome core proteins and class A PBP1a display zonal, processive movement at the midcell of Streptococcus pneumoniae , 2024, 121, 0027-8424, 10.1073/pnas.2401831121 | |
6. | Waldemar Vollmer, 2024, 9780128186190, 45, 10.1016/B978-0-12-818619-0.00015-0 | |
7. | Rinki Gupta, Mangal Singh, Ranjana Pathania, Chemical genetic approaches for the discovery of bacterial cell wall inhibitors, 2023, 14, 2632-8682, 2125, 10.1039/D3MD00143A | |
8. | Miyabi Sakai, Taichi Shimosaka, Kosuke Katsumata, Masafumi Yohda, Issay Narumi, Developing a new host-vector system for Deinococcus grandis, 2024, 15, 1664-302X, 10.3389/fmicb.2024.1387296 | |
9. | Risa Ago, Yuhei O. Tahara, Honoka Yamaguchi, Motoya Saito, Wakana Ito, Kaito Yamasaki, Taishi Kasai, Sho Okamoto, Taiki Chikada, Taku Oshima, Issey Osaka, Makoto Miyata, Hironori Niki, Daisuke Shiomi, Relationship between the Rod complex and peptidoglycan structure in Escherichia coli, 2023, 12, 2045-8827, 10.1002/mbo3.1385 | |
10. | McKenna Harpring, John V. Cox, Plasticity in the cell division processes of obligate intracellular bacteria, 2023, 13, 2235-2988, 10.3389/fcimb.2023.1205488 | |
11. | Sara F. Costa, Bruno M. Saraiva, Helena Veiga, Leonor B. Marques, Simon Schäper, Marta Sporniak, Daniel E. Vega, Ana M. Jorge, Andreia M. Duarte, António D. Brito, Andreia C. Tavares, Patricia Reed, Mariana G. Pinho, N. Luisa Hiller, The role of GpsB in Staphylococcus aureus cell morphogenesis , 2024, 15, 2150-7511, 10.1128/mbio.03235-23 |