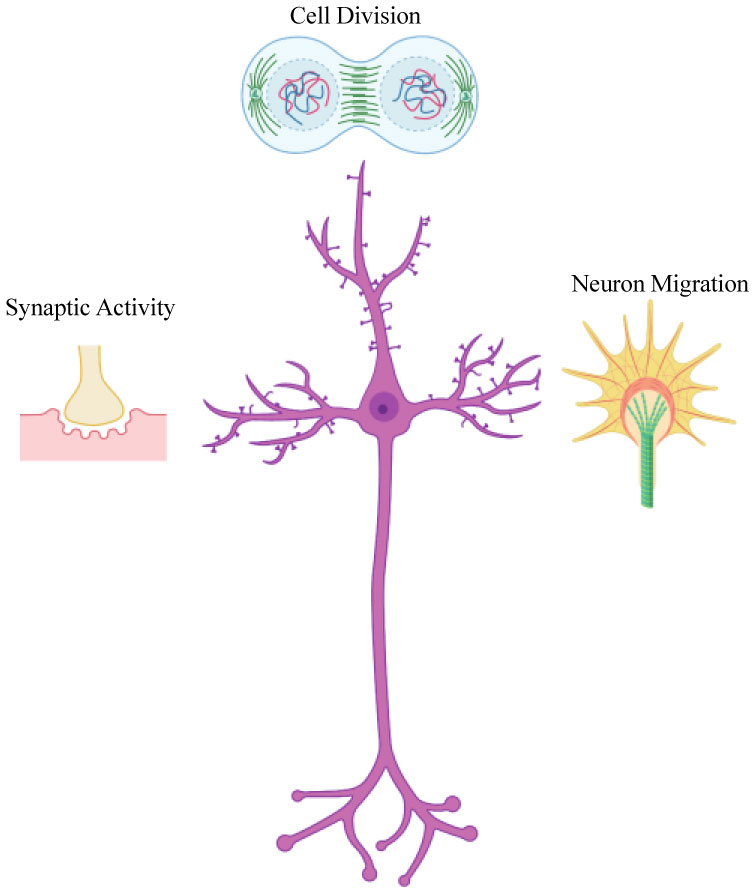
Citation: Mohammad Mofatteh. mRNA localization and local translation in neurons[J]. AIMS Neuroscience, 2020, 7(3): 299-310. doi: 10.3934/Neuroscience.2020016
[1] | Shinichi Tamura, Yoshi Nishitani, Chie Hosokawa . Feasibility of Multiplex Communication in a 2D Mesh Asynchronous Neural Network with Fluctuations. AIMS Neuroscience, 2016, 3(4): 385-397. doi: 10.3934/Neuroscience.2016.4.385 |
[2] | Yoshi Nishitani, Chie Hosokawa, Yuko Mizuno-Matsumoto, Tomomitsu Miyoshi, Shinichi Tamura . Effect of correlating adjacent neurons for identifying communications: Feasibility experiment in a cultured neuronal network. AIMS Neuroscience, 2018, 5(1): 18-31. doi: 10.3934/Neuroscience.2018.1.18 |
[3] | Yang Yu, Tsuyoshi Setogawa, Jumpei Matsumoto, Hiroshi Nishimaru, Hisao Nishijo . Neural basis of topographical disorientation in the primate posterior cingulate gyrus based on a labeled graph. AIMS Neuroscience, 2022, 9(3): 373-394. doi: 10.3934/Neuroscience.2022021 |
[4] | Zinia Pervin, Julia M Stephen . Effect of alcohol on the central nervous system to develop neurological disorder: pathophysiological and lifestyle modulation can be potential therapeutic options for alcohol-induced neurotoxication. AIMS Neuroscience, 2021, 8(3): 390-413. doi: 10.3934/Neuroscience.2021021 |
[5] | Shun Sakuma, Yuko Mizuno-Matsumoto, Yoshi Nishitani, Shinichi Tamura . Learning Times Required to Identify the Stimulated Position and Shortening of Propagation Path by Hebb’s Rule in Neural Network. AIMS Neuroscience, 2017, 4(4): 238-253. doi: 10.3934/Neuroscience.2017.4.238 |
[6] | Yoshi Nishitani, Chie Hosokawa, Yuko Mizuno-Matsumoto, Tomomitsu Miyoshi, Shinichi Tamura . Classification of Spike Wave Propagations in a Cultured Neuronal Network: Investigating a Brain Communication Mechanism. AIMS Neuroscience, 2017, 4(1): 1-13. doi: 10.3934/Neuroscience.2017.1.1 |
[7] | Chacchu Bhattarai, Phanindra Prasad Poudel, Arnab Ghosh, Sneha Guruprasad Kalthur . The RET gene encodes RET protein, which triggers intracellular signaling pathways for enteric neurogenesis, and RET mutation results in Hirschsprung's disease. AIMS Neuroscience, 2022, 9(1): 128-149. doi: 10.3934/Neuroscience.2022008 |
[8] | Yoshi Nishitani, Chie Hosokawa, Yuko Mizuno-Matsumoto, Tomomitsu Miyoshi, Shinichi Tamura . Learning process for identifying different types of communication via repetitive stimulation: feasibility study in a cultured neuronal network. AIMS Neuroscience, 2019, 6(4): 240-249. doi: 10.3934/Neuroscience.2019.4.240 |
[9] | Ernest Greene . New encoding concepts for shape recognition are needed. AIMS Neuroscience, 2018, 5(3): 162-178. doi: 10.3934/Neuroscience.2018.3.162 |
[10] | Jhunlyn Lorenzo, Stéphane Binczak, Sabir Jacquir . A multilayer-multiplexer network processing scheme based on the dendritic integration in a single neuron. AIMS Neuroscience, 2022, 9(1): 76-113. doi: 10.3934/Neuroscience.2022006 |
Since the discovery of polyribosomes at the bases of dendritic spines by Steward and Levy in 1982, many lines of evidence have demonstrated that mRNA localization and de novo synthesis of proteins in the nervous system are critical steps for various nervous system processes such as development of neurons, synaptic plasticity and long-term memory formation [1],[2]. mRNA localization plays a significant role in central and peripheral nervous systems such as neuronal cell division and neuroblast maintenance, neuron migration, and synaptic activity (Figure 1) [3].
Many aspects of neuron behaviour and signalling in the central nervous system (CNS) require spatial control over the distribution of proteins. The positioning of proteins to different regions of the neuron can determine their function. The common depiction in the central dogma of molecular biology that mRNAs are simply transcript molecules for transferring nuclear information in the DNA to the protein synthesis machinery in the cytoplasm has changed. Local control over the expression of genes can be achieved by trafficking mRNA molecules to different subcellular compartments, where they are translated to their protein products. Thus, mRNAs can have active roles in regulating protein expression through their subcellular localization and local translation. However, mRNA trafficking and protein trafficking are not mutually exclusive mechanisms for subcellular localization of cargoes in the cytoplasm. mRNA localization can work in parallel to the protein sorting machinery, ensuring efficient targeting of proteins to their site of action.
The first evidence for asymmetric mRNA localization in the cytoplasm was found in an ascidian, Styela plicata about four decades ago. In situ hybridization using a DNA probe showed that actin mRNA was asymmetrically distributed in different regions of the cytoplasm of the ascidian embryo, with the highest concentration in the myoplasm and the lowest concentration in the endoplasm [4]. Since then, it has been shown that various systems such as Saccharomyces cerevisiae, migrating fibroblasts, Caenorhabditis elegans, Drosophila melanogaster, Xenopus laevis, epithelial cells, neuroblasts, and nerve cells can localize mRNAs [5]–[12]. Observations of asymmetrical distribution of mRNAs in different systems and across different species indicate that subcellular localization of mRNAs is a prevalent and evolutionarily conserved mechanism to distribute proteins to different regions of the cell.
Data from deep sequencing and high-resolution imaging of rat hippocampal neurons led to the conclusion that approximately half of neuronally expressed mRNAs are enriched in dendrites compared to the soma [13]. A significant portion of these mRNAs encode for synaptic proteins such as receptors, scaffolding factors, and proteins involved in signalling pathways.
Localization of mRNA and subsequent translation can be regulated by neuronal activity (Figure 2). Calcium/calmodulin-dependent kinase-2α (CamKIIα) co-localizes in vivo with the RNA localization factor Staufen in dendrites of hippocampal neurons upon depolarisation with potassium chloride (KCl), and the RNA has a stronger enrichment in dendrites upon neuronal stimulation [14]–[16]. β-actin mRNA and its associated proteins can localize in dendritic spines upon neuronal stimulation. Live imaging of a GFP-tagged version of the β-actin mRNA binding protein ZBP1 showed that proteins redistributed from the cell bodies to the base and spines of dendrites of hippocampal neurons upon depolarisation with KCl [17]. The activity-dependent relocalization of ZBP1 was abolished after treatment of neurons with antagonists of NMDA receptors [17]. Also, microtubule-dependent localization of mRNAs encoding the neurotrophin BDNF and its receptor TrkB to dendrites of hippocampal neurons increased two-fold when the neurons were stimulated [18]. Depolarizing cultures of neurons and acute brain slices with KCl elicited a significant increase in the density of β-actin mRNA granules in dendrites [19]. However, it is still unknown whether the activity-dependent increase in mRNA translocation to dendrites is specific to certain mRNA species, or if there is a global increase in the trafficking of mRNAs upon neuron activity. Also, the exact molecular mechanism and potential factors involved in linking neuronal stimulation and mRNA localization are poorly understood.
Bidirectional movement of mRNAs in both anterograde and retrograde can occur in dendrites by simultaneous association of mRNAs with both Dynein and Kinesin. Bidirectional transport of CamKIIα granules can be explained by a study that showed cytoplasmic polyadenylation element binding protein (CPEB) interacts with CamKIIα 3′ UTR and can be co-immunoprecipitated with both Dynein and Kinesin-1 [20]. Overexpression of CPEB enhanced the localization of the mRNA to the dendrites [21], although it was not determined if this mechanism was through a direct effect on Dynein or Kinesin-1 activity.
Molecular motors play a significant role in active localization of mRNAs. The RNA binding protein (RBP) fragile X mental retardation protein (FMRP), which is present in ribonucleoprotein particle (RNP) granules [20], and is involved in regulating translation, can interact with both Kinesin heavy chain (KHC) and Dynein heavy chain (DHC) in Drosophila [22]. FMRP acts as a motor adaptor in mammalian cells, linking Kinesin light chain with mRNA to mediate stimulus-driven mRNA trafficking to dendritic spines [23],[24]. Overexpressing FMRP increased both the frequency and processivity of transporting mRNAs [25]. In contrast, downregulation of FMRP in Drosophila [26] and zebrafish [27] neurons increased arborisation, demonstrating that interfering with this RBP can result in morphological phenotypes. Stationary ARC mRNA molecules have been observed at the base of dendritic spines [28]. The base of dendritic spines can act as a hub for the accumulation of mRNAs, which can move to the spine upon request for local translation. The “sushi belt model” has been put forward to explain the relationship between bidirectional active movements of mRNAs and non-motile mRNAs at the synapses [29]. According to this model, a proportion of mRNAs is constantly moving into and out of the synapse and will be captured upon receiving a stimulus into a spine. Recent live imaging techniques that allow visualisation of translation of individual mRNA molecules in real time [30] can be used to study mRNA localization and translation dynamics in response to neuronal activation.
Coupled with local translation, mRNA localization can provide several advantages compared to protein trafficking. Asymmetric distribution of mRNAs in the cytoplasm can provide neurons with both temporal and spatial resolution over the expression of proteins in different regions of cytoplasm, as translation of pre-localized messages can be triggered on demand. The ability to rapidly respond to different stimuli and regulate the expression level of the corresponding proteins can be crucial in highly polarised cells, such as neurons. Another potential advantage of targeting proteins by prelocalizing their RNAs is that proteins that are newly synthesized from localized mRNAs can have different functions compared to the existing repertoire of proteins in the cell, due to differences in their post-translational modifications (PTMs) [31]. For example, newly synthesised β-actin protein from localizing mRNAs is required for cell polarity and directional movement of fibroblasts [32]. However, the critical molecular distinction between old and new β-actin protein molecules is not known yet and requires further investigation.
In addition, direct transportation of some proteins, especially those that have a high binding affinity to cytoskeletal elements and other cytoplasmic factors, can pose logistically challenging for cells. In such cases, cells can take advantage of localizing translationally silenced mRNAs to their target sites, followed by triggering of translation at the destination. For example, Map2 and Tau proteins bind to microtubules and cannot readily be trafficked to the distal regions of the neurons; therefore, they are localized as precursor mRNAs to dendrites and axons, respectively before they are translated [33]. mRNA localization can be more energy-efficient than direct trafficking of individual proteins. This is because, in principle, multiple proteins can be synthesised from a single localized mRNA on-demand [3]. Finally, locating the elements that mediate mRNA localization in the untranslated regions (UTR) of mRNAs does not require any changes in the coding sequence of transcripts [2]. Thus, there are no additional constraints placed on the sequences used to code the protein.
Formation of a functional neuronal network requires precise positioning of axons in the CNS. Early studies failed to identify ribosomes in mature vertebrates' axons, leading to a notion that all axons do not have the capacity for local translation, and thus shifting the focus to dendritic mRNA trafficking [34]. However, landmark metabolic labelling studies showed that after isolating the soma, developing axons of invertebrates and vertebrates have the capacity to translate mRNAs [35]. Biochemical studies have shown that some of the components of the protein synthesis machinery, such as mRNAs, ribosomal RNAs and translating ribosomes, are present in the giant mature squid axons [36]. Various studies using electron microscopy in vitro and in vivo demonstrated that ribosomes were present in mammalian axons [37],[38]. However, it is still not known whether ribosomes are present in axons as monosomes or polysomes. Data from cultured neurons suggest that ribosomes might form polysomes in cultured cells [37], whereas monosomes might be the predominant species in neurons in vivo [39]. Using immuno-electron microscopy with genetically tagged ribosomes provided ultrastructural evidence for the presence of ribosomes in mature mammalian myelinated in axons was acquired [40].
A study by Tcherkezian et al. [41] could explain the lack of ribosome observation in axons to some extent by showing ribosomes were located very close to the plasma membrane, where they could associate directly with transmembrane (TM) receptors and were released into the axoplasm upon ligand binding and receptor activation. Tethering components of the translation machinery to the cytoplasmic domain of TM receptors allows spatial and temporal regulation of protein expression in response to external cues. Furthermore, electron microscopy of developing Xenopus retinal ganglion cells axons demonstrated that receptor-ribosome coupling is used by guidance cues where guidance cues can induce dissociation of ribosomes followed by specific mRNA translation [42].
For many years it was unclear whether vertebrate axons have the machinery to fold and process the locally synthesised proteins as various electron microscopy attempts failed to identify rough endoplasmic reticulum (RER) and the Golgi apparatus in axons [43]. The failure to identify the RER and the Golgi could be due to morphological properties of axons that are extended in shape. Thus, axons might have structures equivalent to the RER and the Golgi with similar functional properties. Consistent with this notion, fully folded proteins were synthesized and inserted into the plasma membrane in cultured isolated axons in the absence of soma [44]. Similarly, although isolated axons from molluscs (Lymnea stagnalis) do not have conventional RER and Golgi, injection of exogenous mRNAs into axons isolated from the soma resulted in the translation of fully functional transmembrane proteins [45]. Recent proteomic experiments demonstrated that about 350 proteins in isolated retinal axons are dynamically translated in response to different cues [46].
It is still unknown whether newly synthesized proteins in axons require specific post-translation modification compared to the proteins present in the soma, which might be achieved by the presence of RER and Golgi with a special function.
Extrinsic signals can affect growth, pathfinding, and synaptogenesis of developing axons (Figure 3). Mature axons are also dependent on extrinsic factors for maintenance and repair after damage [39]. In vitro data from cultured neurons have shown that isolated axons require axonal protein synthesis and degradation to respond to chemotropic factors or to regenerate after an induced injury (axon-severing) [47],[48]. Developing axons can localize mRNAs and synthesise proteins locally in their growth cones to assist in targeting the correct destination [49],[50].
Navigating axons can also grow in the absence of soma. The removal of the cell body did not affect Xenopus retinal axon pathfinding [52], indicating that translation machinery is available in the axons independent of the soma. Therefore, local protein synthesis in axons can potentially play an important role in both developing and mature axons. Mature axons also have the capacity for mRNA trafficking and protein synthesis for maintenance and regeneration after injury [49],[50].
Microarray analysis combined with fluorescent in situ hybridization (FISH) showed that more than 300 mRNAs encoding various components of the translational machinery, cargo transportation, cytoskeleton and mitochondria are enriched in axons of the CNS and peripheral nervous system (PNS) of mammalian neurons [50]. In their study, healthy axons were capable of changing the composition of localizing mRNAs following axotomy by enriching mRNAs required for repair and regeneration [50]. While mRNAs encoding components of cargo trafficking, cytoskeleton and mitochondria showed a decrease in localization, there was an upregulation in localization of transcripts encoding proteins involved in axon targeting and synaptogenesis. These observations provided evidence for RNA localization-based adaptation of injured axons to regenerate and form synapses (Figure 4).
Axonal branching in the vertebrate CNS is linked closely to local protein synthesis. Guidance cues such as Netrin-1, BDNF, Sema3A, and Slit2 trigger local protein synthesis in the responsive neuron [48],[51],[53], and also function as modulators of arborisation [54]. For instance, the stimulus-dependent phosphorylation of the ZBP1 RBP by Src in response to BDNF resulted in ZBP1 dissociation from β-actin and translation induction, thereby promoting growth cone turning [55].
In cultured dorsal root ganglion (DRG) neurons, mRNAs encoding the components of the actin-nucleating Arp2/3 complex are localized to branch points and promote branch formation in response to nerve growth factor (NGF) [56]. Using high-resolution live imaging, it was shown that RNA granules localized to the sites of nascent branch formation in retinal ganglion cell (RGC) neurons in Xenopus in vivo, and move to the distal tip of stable branches [57]. Blocking β-actin translation disrupted branch formation, thereby demonstrating the importance of mRNA localization and local protein synthesis in arbour formation and stabilisation [57]. Thus, local synthesis of components of the actin cytoskeleton can provide the basis for branch formation on-demand. Interestingly, quantitative proteomic studies demonstrated that Arp2/3 complex, WAVE2, VASP and other actin binding molecules are enriched in cell protrusions, a role in agreement with their involvement in neuronal branch formation [58].
Deep sequencing of ribosome-bound mRNAs showed that many presynaptic proteins are translated locally in mouse retinal axons during branch formation [59]. The degree of presynaptic arborisation can determine the number of post-synaptic connections that a neuron can have, hence defining the complexity of neural circuits formation in the CNS [60],[61]. Hence, local protein synthesis in axons can have a fundamental role in synaptogenesis and neural circuit assembly.
Defective assembly of RNPs has been implicated in the pathogenesis of neurological diseases that affect axons such as amyotrophic lateral sclerosis (ALS) and spinal muscular atrophy (SMA) [62]. Survival motor neuron (SMN) protein regulates localization and local translation of β-actin, growth-associated protein 43 (GAP43) and neuritin/cpg15 mRNAs in motor neurons [62],[63]–[68]. Overexpression of SMN-interacting proteins HuD and ZBP1 rescued the reduced translation of the interacting mRNAs in the growth cone [68]. Thus, there is compelling evidence that mRNA trafficking in axons plays an important role in axonal development, maintenance and disease. However, the mechanisms involved in linking axonal mRNAs to molecular motors and the RNA localization signals required for axonal mRNA targeting are still poorly understood. A mechanistic understanding of RNA localization in axons may provide us with information on mechanisms of neurological diseases.
Localization of mRNA and subsequent translation can provide intricate network of the neurons with a delicate control over gene expression in different compartments at different times. Various studies have demonstrated the functional importance of mRNA localization in axon and dendrites. While molecular mechanism of mRNA localization is conserved in neurons from invertebrates to vertebrates, the role of different factors and regulators in trafficking and translation of mRNAs remains to be discovered. Although in vitro studies of cultured neurons have provided very useful information on mRNA localisation and local translation, we still do not have a clear understanding of the detailed molecular mechanisms underlying axonal mRNA localisation. This is particularly the case in vivo, where neurons are present in their physiological environment. Further studies are required to decipher the role of neuronal activity in mRNA localization during formation of neuronal network.
[1] |
Mofatteh M, Bullock SL (2017) SnapShot: Subcellular mRNA localization. Cell 169: 178-178.e1. doi: 10.1016/j.cell.2017.03.004
![]() |
[2] |
Holt CE, Schuman EM (2013) The central dogma decentralized: New perspectives on RNA function and local translation in neurons. Neuron 80: 648-657. doi: 10.1016/j.neuron.2013.10.036
![]() |
[3] |
Buxbaum AR, Haimovich G, Singer RH (2015) In the right place at the right time: visualizing and understanding mRNA localization. Nat Rev Mol Cell Biol 16: 95-109. doi: 10.1038/nrm3918
![]() |
[4] |
Jeffery WR, Tomlinson CR, Brodeur RD (1983) Localization of actin messenger RNA during early ascidian development. Dev Biol 99: 408-417. doi: 10.1016/0012-1606(83)90290-7
![]() |
[5] |
Long RM, Singer RH, Meng X, et al. (1997) Mating type switching in yeast controlled by asymmetric localization of ASH1 mRNA. Science 277: 383-387. doi: 10.1126/science.277.5324.383
![]() |
[6] |
Lawrence JB, Singer RH (1986) Intracellular localization of messenger RNAs for cytoskeletal proteins. Cell 45: 407-415. doi: 10.1016/0092-8674(86)90326-0
![]() |
[7] | Tabara H, Hill RJ, Mello CC, et al. (1999) pos-1 encodes a cytoplasmic zinc-finger protein essential for germline specification in C. elegans. Development 126: 1-11. |
[8] |
Berleth T, Burri M, Thoma G, et al. (1988) The role of localization of bicoid RNA in organizing the anterior pattern of the Drosophila embryo. EMBO J 7: 1749-1756. doi: 10.1002/j.1460-2075.1988.tb03004.x
![]() |
[9] |
Melton DA (1987) Translocation of a localized maternal mRNA to the vegetal pole of Xenopus oocytes. Nature 328: 80-82. doi: 10.1038/328080a0
![]() |
[10] |
Houle VM, Li W, Montgomery RK, et al. (2003) mRNA localization in polarized intestinal epithelial cells. Am J Physiol Gastrointest Liver Physiol 284: G722-G727. doi: 10.1152/ajpgi.00458.2002
![]() |
[11] |
Broadus J, Fuerstenberg S, Doe CQ (1998) Staufen-dependent localization of prospero mRNA contributes to neuroblast daughter-cell fate. Nature 391: 792-795. doi: 10.1038/35861
![]() |
[12] |
Garner CC, Tucker RP, Matus A (1988) Selective localization of messenger RNA for cytoskeletal protein MAP2 in dendrites. Nature 336: 674-677. doi: 10.1038/336674a0
![]() |
[13] |
Cajigas IJ, Tushev G, Will TJ, et al. (2012) The local transcriptome in the synaptic neuropil revealed by deep sequencing and high-resolution imaging. Neuron 74: 453-466. doi: 10.1016/j.neuron.2012.02.036
![]() |
[14] |
Köhrmann M, Luo M, Kaether C, et al. (1999) Microtubule-dependent recruitment of Staufen-green fluorescent protein into large RNA-containing granules and subsequent dendritic transport in living hippocampal neurons. Mol Biol Cell 10: 2945-2953. doi: 10.1091/mbc.10.9.2945
![]() |
[15] |
Mikl M, Vendra G, Kiebler MA (2011) Independent localization of MAP2, CaMKIIα and β-actin RNAs in low copy numbers. EMBO Rep 12: 1077-1084. doi: 10.1038/embor.2011.149
![]() |
[16] |
Roos J, Hummel T, Ng N, et al. (2000) Drosophila futsch regulates synaptic microtubule organization and is necessary for synaptic growth. Neuron 26: 371-382. doi: 10.1016/S0896-6273(00)81170-8
![]() |
[17] |
Tiruchinapalli DM, Oleynikov Y, Kelic S, et al. (2003) Activity-dependent trafficking and dynamic localization of zipcode binding protein 1 and beta-actin mRNA in dendrites and spines of hippocampal neurons. J Neurosci 23: 3251-3261. doi: 10.1523/JNEUROSCI.23-08-03251.2003
![]() |
[18] |
Tongiorgi E, Armellin M, Giulianini PG, et al. (2004) Brain-derived neurotrophic factor mRNA and protein are targeted to discrete dendritic laminas by events that trigger epileptogenesis. J Neurosci 24: 6842-6852. doi: 10.1523/JNEUROSCI.5471-03.2004
![]() |
[19] |
Park HY, Lim H, Yoon YJ, et al. (2014) Visualization of dynamics of single endogenous mRNA labeled in live mouse. Science 343: 422-424. doi: 10.1126/science.1239200
![]() |
[20] |
Kanai Y, Dohmae N, Hirokawa N (2004) Kinesin transports RNA: Isolation and characterization of an RNA-Transporting granule. Neuron 43: 513-525. doi: 10.1016/j.neuron.2004.07.022
![]() |
[21] |
Rook MS, Lu M, Kosik KS (2000) CaMKIIalpha 3′ untranslated region-directed mRNA translocation in living neurons: visualization by GFP linkage. J Neurosci 20: 6385-6393. doi: 10.1523/JNEUROSCI.20-17-06385.2000
![]() |
[22] |
Ling SC, Fahrner PS, Greenough WT, et al. (2004) Transport of Drosophila fragile X mental retardation protein-containing ribonucleoprotein granules by kinesin-1 and cytoplasmic dynein. Proc Natl Acad Sci 101: 17428-17433. doi: 10.1073/pnas.0408114101
![]() |
[23] |
Dictenberg JB, Swanger SA, Antar LN, et al. (2008) A direct role for FMRP in activity-dependent dendritic mRNA transport links filopodial-spine morphogenesis to fragile X syndrome. Dev Cell 14: 926-939. doi: 10.1016/j.devcel.2008.04.003
![]() |
[24] |
Kao DI, Aldridge GM, Weiler IJ, et al. (2010) Altered mRNA transport, docking, and protein translation in neurons lacking fragile X mental retardation protein. Proc Natl Acad Sci 107: 15601-15606. doi: 10.1073/pnas.1010564107
![]() |
[25] |
Estes PS, O'Shea M, Clasen S, et al. (2008) Fragile X protein controls the efficacy of mRNA transport in Drosophila neurons. Mol Cell Neurosci 39: 170-179. doi: 10.1016/j.mcn.2008.06.012
![]() |
[26] |
Pan L, Zhang YQ, Woodruff E, et al. (2004) The Drosophila fragile X gene negatively regulates neuronal elaboration and synaptic differentiation. Curr Biol 14: 1863-1870. doi: 10.1016/j.cub.2004.09.085
![]() |
[27] |
Tucker B, Richards RI, Lardelli M (2006) Contribution of mGluR and Fmr1 functional pathways to neurite morphogenesis, craniofacial development and fragile X syndrome. Hum Mol Genet 15: 3446-3458. doi: 10.1093/hmg/ddl422
![]() |
[28] |
Dynes JL, Steward O (2007) Dynamics of bidirectional transport of Arc mRNA in neuronal dendrites. J Comp Neurol 500: 433-447. doi: 10.1002/cne.21189
![]() |
[29] |
Doyle M, Kiebler MA (2011) Mechanisms of dendritic mRNA transport and its role in synaptic tagging. EMBO J 30: 3540-3552. doi: 10.1038/emboj.2011.278
![]() |
[30] |
Wang C, Han B, Zhou R, et al. (2016) Real-time imaging of translation on single mRNA transcripts in live cells. Cell 165: 990-1001. doi: 10.1016/j.cell.2016.04.040
![]() |
[31] |
Weatheritt RJ, Gibson TJ, Babu MM (2014) Asymmetric mRNA localization contributes to fidelity and sensitivity of spatially localized systems. Nat Struct Mol Biol 21: 833-839. doi: 10.1038/nsmb.2876
![]() |
[32] |
Liao G, Mingle L, Van De Water L, et al. (2015) Control of cell migration through mRNA localization and local translation. Wiley Interdiscip Rev RNA 6: 1-15. doi: 10.1002/wrna.1265
![]() |
[33] |
Park HY, Trcek T, Wells AL, et al. (2012) An unbiased analysis method to quantify mRNA localization reveals its correlation with cell motility. Cell Rep 1: 179-184. doi: 10.1016/j.celrep.2011.12.009
![]() |
[34] |
Twiss JL, Fainzilber M (2009) Ribosomes in axons- scrounging from the neighbors? Trends Cell Biol 19: 236-243. doi: 10.1016/j.tcb.2009.02.007
![]() |
[35] |
Eng H, Lund K, Campenot RB (1999) Synthesis of β-Tubulin, actin, and other proteins in axons of sympathetic neurons in compartmented cultures. J Neurosci 19: 1-9. doi: 10.1523/JNEUROSCI.19-01-00001.1999
![]() |
[36] |
Giuditta A, Menichini E, Capano CP, et al. (1991) Active polysomes in the axoplasm of the squid giant axon. J Neurosci Res 28: 18-28. doi: 10.1002/jnr.490280103
![]() |
[37] |
Bassell GJ, Zhang H, Byrd AL, et al. (1998) Sorting of beta-actin mRNA and protein to neurites and growth cones in culture. J Neurosci 18: 251-265. doi: 10.1523/JNEUROSCI.18-01-00251.1998
![]() |
[38] |
Bunge MB (1973) Fine structure of nerve fibers and growth cones of isolated sympathetic neurons in culture. J Cell Biol 56: 713-735. doi: 10.1083/jcb.56.3.713
![]() |
[39] |
Jung H, Yoon BC, Holt CE (2012) Axonal mRNA localization and local protein synthesis in nervous system assembly, maintenance and repair. Nat Rev Neurosci 13: 308-324. doi: 10.1038/nrn3210
![]() |
[40] |
Shigeoka T, Jung H, Jung J, et al. (2016) Dynamic axonal translation in developing and mature visual circuits. Cell 166: 181-192. doi: 10.1016/j.cell.2016.05.029
![]() |
[41] |
Tcherkezian J, Brittis PA, Thomas F, et al. (2010) Transmembrane receptor DCC associates with protein synthesis machinery and regulates translation. Cell 141: 632-644. doi: 10.1016/j.cell.2010.04.008
![]() |
[42] |
Koppers M, Cagnetta R, Shigeoka T, et al. (2019) Receptor-specific interactome as a hub for rapid cue-induced selective translation in axons. eLife 8: e48718. doi: 10.7554/eLife.48718
![]() |
[43] |
Zheng JQ, Kelly TK, Chang B, et al. (2001) A functional role for intra-axonal protein synthesis during axonal regeneration from adult sensory neurons. J Neurosci 21: 9291-9303. doi: 10.1523/JNEUROSCI.21-23-09291.2001
![]() |
[44] |
Merianda TT, Lin AC, Lam JSY, et al. (2009) A functional equivalent of endoplasmic reticulum and Golgi in axons for secretion of locally synthesized proteins. Mol Cell Neurosci 40: 128-142. doi: 10.1016/j.mcn.2008.09.008
![]() |
[45] |
Spencer GE, Syed NI, van Kesteren E, et al. (2000) Synthesis and functional integration of a neurotransmitter receptor in isolated invertebrate axons. J Neurobiol 44: 72-81. doi: 10.1002/1097-4695(200007)44:1<72::AID-NEU7>3.0.CO;2-#
![]() |
[46] |
Cagnetta R, Frese CK, Shigeoka T, et al. (2018) Rapid cue-specific remodeling of the nascent axonal proteome. Neuron 99: 29-46. e4. doi: 10.1016/j.neuron.2018.06.004
![]() |
[47] |
Verma P, Chierzi S, Codd AM, et al. (2005) Axonal protein synthesis and degradation are necessary for efficient growth cone regeneration. J Neurosci 25: 331-342. doi: 10.1523/JNEUROSCI.3073-04.2005
![]() |
[48] |
Campbell DS, Holt CE (2001) Chemotropic responses of retinal growth cones mediated by rapid local protein synthesis and degradation. Neuron 32: 1013-1026. doi: 10.1016/S0896-6273(01)00551-7
![]() |
[49] |
Andreassi C, Zimmermann C, Mitter R, et al. (2010) An NGF-responsive element targets myo-inositol monophosphatase-1 mRNA to sympathetic neuron axons. Nat Neurosci 13: 291-301. doi: 10.1038/nn.2486
![]() |
[50] |
Zivraj KH, Tung YCL, Piper M, et al. (2010) Subcellular profiling reveals distinct and developmentally regulated repertoire of growth cone mRNAs. J Neurosci 30: 15464-15478. doi: 10.1523/JNEUROSCI.1800-10.2010
![]() |
[51] |
Lin AC, Holt CE (2007) Local translation and directional steering in axons. EMBO J 26: 3729-3736. doi: 10.1038/sj.emboj.7601808
![]() |
[52] | Harris WA, Holt CE, Bonhoeffer F (1987) Retinal axons with and without their somata, growing to and arborizing in the tectum of Xenopus embryos: a time-lapse video study of single fibres in vivo. Development 101: 123-133. |
[53] |
Sahoo PK, Smith DS, Perrone-Bizzozero N, et al. (2018) Axonal mRNA transport and translation at a glance. J Cell Sci 131: jcs196808. doi: 10.1242/jcs.196808
![]() |
[54] |
Cosker KE, Fenstermacher SJ, Pazyra-Murphy MF, et al. (2016) The RNA-binding protein SFPQ orchestrates an RNA regulon to promote axon viability. Nat Neurosci 19: 690-696. doi: 10.1038/nn.4280
![]() |
[55] |
Sasaki Y, Welshhans K, Wen Z, et al. (2010) Phosphorylation of zipcode binding protein 1 is required for brain-derived neurotrophic factor signaling of local beta-actin synthesis and growth cone turning. J Neurosci 30: 9349-9358. doi: 10.1523/JNEUROSCI.0499-10.2010
![]() |
[56] |
Spillane M, Ketschek A, Donnelly CJ, et al. (2012) Nerve growth factor-induced formation of axonal filopodia and collateral branches involves the intra-axonal synthesis of regulators of the actin-nucleating Arp2/3 complex. J Neurosci 32: 17671-17689. doi: 10.1523/JNEUROSCI.1079-12.2012
![]() |
[57] |
Wong HHW, Lin JQ, Ströhl F, et al. (2017) RNA docking and local translation regulate site-specific axon remodeling in vivo. Neuron 95: 852-868. e8. doi: 10.1016/j.neuron.2017.07.016
![]() |
[58] |
Mardakheh FK, Paul A, Kümper S, et al. (2015) Global analysis of mRNA, translation, and protein localization: Local translation is a key regulator of cell protrusions. Dev Cell 35: 344-357. doi: 10.1016/j.devcel.2015.10.005
![]() |
[59] |
Shigeoka T, Jung H, Jung J, et al. (2016) Dynamic axonal translation in developing and mature visual circuits. Cell 166: 181-192. doi: 10.1016/j.cell.2016.05.029
![]() |
[60] |
Piper M, Anderson R, Dwivedy A, et al. (2006) Signaling mechanisms underlying Slit2-induced collapse of Xenopus retinal growth cones. Neuron 49: 215-228. doi: 10.1016/j.neuron.2005.12.008
![]() |
[61] |
Meyer MP, Smith SJ (2006) Evidence from in vivo imaging that synaptogenesis guides the growth and branching of axonal arbors by two distinct mechanisms. J Neurosci 26: 3604-3614. doi: 10.1523/JNEUROSCI.0223-06.2006
![]() |
[62] |
Sanchez G, Dury AY, Murray LM, et al. (2012) A novel function for the survival motoneuron protein as a translational regulator. Hum Mol Genet 22: 668-684. doi: 10.1093/hmg/dds474
![]() |
[63] |
Shukla S, Parker R (2016) Hypo- and Hyper-Assembly diseases of RNA-Protein complexes. Trends Mol Med 22: 615-628. doi: 10.1016/j.molmed.2016.05.005
![]() |
[64] |
Jung H, Yoon BC, Holt CE (2012) Axonal mRNA localization and local protein synthesis in nervous system assembly, maintenance and repair. Nat Rev Neurosci 13: 308-324. doi: 10.1038/nrn3210
![]() |
[65] |
Hao le T, Duy PQ, An M, et al. (2017) HuD and the survival motor neuron protein interact in motoneurons and are essential for motoneuron development, function, and mRNA regulation. J Neurosci 37: 11559-11571. doi: 10.1523/JNEUROSCI.1528-17.2017
![]() |
[66] |
Fallini C, Zhang H, Su Y, et al. (2011) The survival of motor neuron (SMN) protein interacts with the mRNA-binding protein HuD and regulates localization of poly(A) mRNA in primary motor neuron axons. J Neurosci 31: 3914-3925. doi: 10.1523/JNEUROSCI.3631-10.2011
![]() |
[67] |
Akten B, Kye MJ, Hao LT, et al. (2011) Interaction of survival of motor neuron (SMN) and HuD proteins with mRNA cpg15 rescues motor neuron axonal deficits. Proc Natl Acad Sci 108: 10337-10342. doi: 10.1073/pnas.1104928108
![]() |
[68] |
Fallini C, Donlin-Asp PG, Rouanet JP, et al. (2016) Deficiency of the survival of motor neuron protein impairs mRNA localization and local translation in the growth cone of motor neurons. J Neurosci 36: 3811-3820. doi: 10.1523/JNEUROSCI.2396-15.2016
![]() |
1. | Yuyoung Joo, David R. Benavides, Local Protein Translation and RNA Processing of Synaptic Proteins in Autism Spectrum Disorder, 2021, 22, 1422-0067, 2811, 10.3390/ijms22062811 | |
2. | Mohammad Mofatteh, Neurosurgery and artificial intelligence, 2021, 8, 2373-7972, 477, 10.3934/Neuroscience.2021025 | |
3. | Lisa Pavinato, Andrea Delle Vedove, Diana Carli, Marta Ferrero, Silvia Carestiato, Jennifer L Howe, Emanuele Agolini, Domenico A Coviello, Ingrid van de Laar, Ping Yee Billie Au, Eleonora Di Gregorio, Alessandra Fabbiani, Susanna Croci, Maria Antonietta Mencarelli, Lucia P Bruno, Alessandra Renieri, Danai Veltra, Christalena Sofocleous, Laurence Faivre, Benoit Mazel, Hana Safraou, Anne-Sophie Denommé-Pichon, Marjon A van Slegtenhorst, Noor Giesbertz, Richard H van Jaarsveld, Anna Childers, R Curtis Rogers, Antonio Novelli, Silvia De Rubeis, Joseph D Buxbaum, Stephen W Scherer, Giovanni Battista Ferrero, Brunhilde Wirth, Alfredo Brusco, CAPRIN1 haploinsufficiency causes a neurodevelopmental disorder with language impairment, ADHD and ASD, 2023, 146, 0006-8950, 534, 10.1093/brain/awac278 | |
4. | Sudhriti Ghosh Dastidar, Deepak Nair, A Ribosomal Perspective on Neuronal Local Protein Synthesis, 2022, 15, 1662-5099, 10.3389/fnmol.2022.823135 | |
5. | Nikoletta Triantopoulou, Marina Vidaki, Local mRNA translation and cytoskeletal reorganization: Mechanisms that tune neuronal responses, 2022, 15, 1662-5099, 10.3389/fnmol.2022.949096 | |
6. | Paola Bonsi, Antonella De Jaco, Laurent Fasano, Paolo Gubellini, Postsynaptic autism spectrum disorder genes and synaptic dysfunction, 2022, 162, 09699961, 105564, 10.1016/j.nbd.2021.105564 | |
7. | Jianqing Wu, John Akighir, FDA Should Re-evaluate All mRNA Vaccines and Revoke Their Use Authorizations (The Short Version)., 2022, 4, 26921537, 16, 10.14302/issn.2692-1537.ijcv-21-4053 | |
8. | Ligia B. Schmitd, Cindy Perez-Pacheco, Emily L. Bellile, Weisheng Wu, Keith Casper, Michelle Mierzwa, Laura S. Rozek, Gregory T. Wolf, Jeremy M.G. Taylor, Nisha J. D'Silva, Spatial and Transcriptomic Analysis of Perineural Invasion in Oral Cancer, 2022, 28, 1078-0432, 3557, 10.1158/1078-0432.CCR-21-4543 | |
9. | Josephine A. Carew, Vivian Cristofaro, Raj K. Goyal, Maryrose P. Sullivan, Differential Myosin 5a splice variants in innervation of pelvic organs, 2023, 14, 1664-042X, 10.3389/fphys.2023.1304537 | |
10. | Mohammad Mofatteh, Mohammad Sadegh Mashayekhi, Saman Arfaie, Yimin Chen, Armaan K. Malhotra, Georgios P. Skandalakis, Mohammed Ali Alvi, Fardad T. Afshari, Shakila Meshkat, Famu Lin, Ebtesam Abdulla, Ayush Anand, Xuxing Liao, Roger S. McIntyre, Carlo Santaguida, Michael H. Weber, Michael G. Fehlings, Anxiety and Depression in Pediatric-Onset Traumatic Spinal Cord Injury: A Systematic Review, 2024, 184, 18788750, 267, 10.1016/j.wneu.2023.12.092 | |
11. | Julia Olivares-Abril, Jana Joha, Jeffrey Y. Lee, Ilan Davis, Optimization of hybridization chain reaction for imaging single RNA molecules in Drosophila larvae , 2024, 18, 1933-6934, 10.1080/19336934.2024.2409968 | |
12. | Wen Xiao, Reem Halabi, Chia-Ho Lin, Mohammad Nazim, Kyu-Hyeon Yeom, Douglas L. Black, The lncRNAMalat1is trafficked to the cytoplasm as a localized mRNA encoding a small peptide in neurons, 2024, 38, 0890-9369, 294, 10.1101/gad.351557.124 | |
13. | Dennis Quentin, Jan S. Schuhmacher, Björn U. Klink, Jeni Lauer, Tanvir R. Shaikh, Pim J. Huis in ’t Veld, Luisa M. Welp, Henning Urlaub, Marino Zerial, Stefan Raunser, Structural basis of mRNA binding by the human FERRY Rab5 effector complex, 2023, 83, 10972765, 1856, 10.1016/j.molcel.2023.05.009 | |
14. | Hikari Nishisaka, Takumi Tomohiro, Kako Fukuzumi, Akira Fukao, Yoshinori Funakami, Toshinobu Fujiwara, Deciphering the Akt1-HuD interaction in HuD-mediated neuronal differentiation, 2024, 221, 03009084, 20, 10.1016/j.biochi.2024.01.010 | |
15. | Kara Snyder, C. Edward Dixon, Jeremy Henchir, Kiersten Gorse, Vincent A. Vagni, Keri Janesko-Feldman, Patrick M. Kochanek, Travis C. Jackson, Gene knockout of RNA binding motif 5 in the brain alters RIMS2 protein homeostasis in the cerebellum and Hippocampus and exacerbates behavioral deficits after a TBI in mice, 2024, 374, 00144886, 114690, 10.1016/j.expneurol.2024.114690 | |
16. | Zhonghao Yu, Yue Guan, Tian Xia, Xuanwen Li, Mingyue Liu, Yujia Huo, Zhuowei Wang, Zhirong Liu, Yuting Luo, Wentao Yan, Lanfang Sun, Wencan Wu, Baoguo Shen, Yikui Zhang, Overcoming Low mRNA Expression in White Matter: A Protocol for RNA Extraction From the Optic Nerve in Large Animals for Transcriptomic Analysis, 2024, 65, 1552-5783, 25, 10.1167/iovs.65.13.25 | |
17. | Mohammad Mofatteh, Examining the association between traumatic brain injury and headache, 2021, 20, 0219-6352, 10.31083/j.jin2004109 | |
18. | Pargol Tayefeh Ghahremani, Soha BaniArdalan, Parsa Alehossein, Arshi Parveen, Masoumeh Jorjani, Candice M. Brown, Werner J. Geldenhuys, Jason D. Huber, Tauheed Ishrat, Sanaz Nasoohi, Poststroke hyperglycemia dysregulates cap-dependent translation in neural cells, 2025, 361, 00243205, 123336, 10.1016/j.lfs.2024.123336 | |
19. | Alexandria L. Quillin, Diane B. Karloff, Tewoderos M. Ayele, Tatiana F. Flores, Gerry Chen, Zachary T. McEachin, Arielle N. Valdez-Sinon, Jennifer M. Heemstra, Imaging and Tracking RNA in Live Mammalian Cells via Fluorogenic Photoaffinity Labeling, 2025, 1554-8929, 10.1021/acschembio.4c00848 | |
20. | Vanessa Lakis, Noni L Chan, Ruth Lyons, Nicola Blackburn, Tam Hong Nguyen, Crystal Chang, Andrew Masel, Nicholas P. West, Glen M. Boyle, Ann-Marie Patch, Anthony J. Gill, Katia Nones, Spatial Transcriptomics Reveals Novel Mechanisms Involved in Perineural Invasion in Pancreatic Ductal Adenocarcinomas, 2025, 17, 2072-6694, 852, 10.3390/cancers17050852 | |
21. | David Zimmermann, Michaela Kress, Istvan Nagy, Established and emerging roles of protein kinases in regulating primary sensory neurons in injury-and inflammation-associated pain, 2025, 1472-8222, 10.1080/14728222.2025.2489540 | |
22. | Dennis J Hazelett, Jonathan Wren, Rethinking GWAS: how lessons from genetic screens and artificial intelligence could reveal biological mechanisms, 2025, 41, 1367-4811, 10.1093/bioinformatics/btaf153 | |
23. | Riya Thomas, Die Zhang, Christopher A. Cronkite, Rintu Thomas, Sanjay K. Singh, Lawrence F. Bronk, Rodrigo F. Morales, Joseph G. Duman, David R. Grosshans, Subcellular functions of tau mediate repair response and synaptic homeostasis in injury, 2025, 1359-4184, 10.1038/s41380-025-03029-6 |