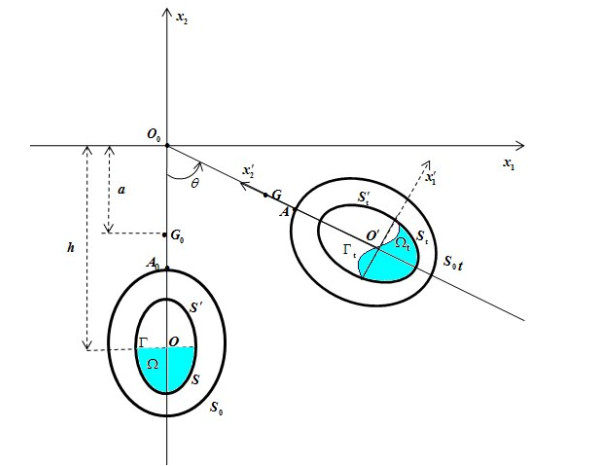
This study mainly used The Cancer Genome Atlas (TCGA) RNA sequencing dataset to screen prognostic snoRNAs of acute myeloid leukemia (AML), and used for the construction of prognostic snoRNAs signature for AML. A total of 130 AML patients with RNA sequencing dataset were used for prognostic snoRNAs screenning. SnoRNAs co-expressed genes and differentially expressed genes (DEGs) were used for functional annotation, as well as gene set enrichment analysis (GSEA). Connectivity Map (CMap) also used for potential targeted drugs screening. Through genome-wide screening, we identified 30 snoRNAs that were significantly associated with the prognosis of AML. Then we used the step function to screen a prognostic signature composed of 14 snoRNAs (SNORD72, SNORD38, U3, SNORA73B, SNORD79, SNORA73, SNORD12B, SNORA74, SNORD116-12, SNORA65, SNORA14, snoU13, SNORA75, SNORA31), which can significantly divide AML patients into high- and low-risk groups. Through GSEA, snoRNAs co-expressed genes and DEGs functional enrichment analysis, we screened a large number of potential functional mechanisms of this prognostic signature in AML, such as phosphatidylinositol 3-kinase-Akt, Wnt, epithelial to mesenchymal transition, T cell receptors, NF-kappa B, mTOR and other classic cancer-related signaling pathways. In the subsequent targeted drug screening using CMap, we also identified six drugs that can be used for AML targeted therapy, they were alimemazine, MG-262, fluoxetine, quipazine, naltrexone and oxybenzone. In conclusion, our current study was constructed an AML prognostic signature based on the 14 prognostic snoRNAs, which may serve as a novel prognostic biomarker for AML.
Citation: Rui Huang, Xiwen Liao, Qiaochuan Li. Integrative genomic analysis of a novel small nucleolar RNAs prognostic signature in patients with acute myelocytic leukemia[J]. Mathematical Biosciences and Engineering, 2022, 19(3): 2424-2452. doi: 10.3934/mbe.2022112
[1] | Yang Liu, Xiao Long, Li Zhang . Long-time dynamics for a coupled system modeling the oscillations of suspension bridges. Communications in Analysis and Mechanics, 2025, 17(1): 15-40. doi: 10.3934/cam.2025002 |
[2] | Ying Chu, Bo Wen, Libo Cheng . Existence and blow up for viscoelastic hyperbolic equations with variable exponents. Communications in Analysis and Mechanics, 2024, 16(4): 717-737. doi: 10.3934/cam.2024032 |
[3] | Katica R. (Stevanović) Hedrih, Gradimir V. Milovanović . Elements of mathematical phenomenology and analogies of electrical and mechanical oscillators of the fractional type with finite number of degrees of freedom of oscillations: linear and nonlinear modes. Communications in Analysis and Mechanics, 2024, 16(4): 738-785. doi: 10.3934/cam.2024033 |
[4] | Haifa El Jarroudi, Mustapha El Jarroudi . Asymptotic behavior of a viscous incompressible fluid flow in a fractal network of branching tubes. Communications in Analysis and Mechanics, 2024, 16(3): 655-699. doi: 10.3934/cam.2024030 |
[5] | Hongxia Lin, Sabana, Qing Sun, Ruiqi You, Xiaochuan Guo . The stability and decay of 2D incompressible Boussinesq equation with partial vertical dissipation. Communications in Analysis and Mechanics, 2025, 17(1): 100-127. doi: 10.3934/cam.2025005 |
[6] | Leandro Tavares . Solutions for a class of problems driven by an anisotropic (p,q)-Laplacian type operator. Communications in Analysis and Mechanics, 2023, 15(3): 533-550. doi: 10.3934/cam.2023026 |
[7] | Yao Sun, Pan Wang, Xinru Lu, Bo Chen . A boundary integral equation method for the fluid-solid interaction problem. Communications in Analysis and Mechanics, 2023, 15(4): 716-742. doi: 10.3934/cam.2023035 |
[8] | Yang Liu . Global attractors for a nonlinear plate equation modeling the oscillations of suspension bridges. Communications in Analysis and Mechanics, 2023, 15(3): 436-456. doi: 10.3934/cam.2023021 |
[9] | Long Ju, Jian Zhou, Yufeng Zhang . Conservation laws analysis of nonlinear partial differential equations and their linear soliton solutions and Hamiltonian structures. Communications in Analysis and Mechanics, 2023, 15(2): 24-49. doi: 10.3934/cam.2023002 |
[10] | Ho-Sik Lee, Youchan Kim . Boundary Riesz potential estimates for parabolic equations with measurable nonlinearities. Communications in Analysis and Mechanics, 2025, 17(1): 61-99. doi: 10.3934/cam.2025004 |
This study mainly used The Cancer Genome Atlas (TCGA) RNA sequencing dataset to screen prognostic snoRNAs of acute myeloid leukemia (AML), and used for the construction of prognostic snoRNAs signature for AML. A total of 130 AML patients with RNA sequencing dataset were used for prognostic snoRNAs screenning. SnoRNAs co-expressed genes and differentially expressed genes (DEGs) were used for functional annotation, as well as gene set enrichment analysis (GSEA). Connectivity Map (CMap) also used for potential targeted drugs screening. Through genome-wide screening, we identified 30 snoRNAs that were significantly associated with the prognosis of AML. Then we used the step function to screen a prognostic signature composed of 14 snoRNAs (SNORD72, SNORD38, U3, SNORA73B, SNORD79, SNORA73, SNORD12B, SNORA74, SNORD116-12, SNORA65, SNORA14, snoU13, SNORA75, SNORA31), which can significantly divide AML patients into high- and low-risk groups. Through GSEA, snoRNAs co-expressed genes and DEGs functional enrichment analysis, we screened a large number of potential functional mechanisms of this prognostic signature in AML, such as phosphatidylinositol 3-kinase-Akt, Wnt, epithelial to mesenchymal transition, T cell receptors, NF-kappa B, mTOR and other classic cancer-related signaling pathways. In the subsequent targeted drug screening using CMap, we also identified six drugs that can be used for AML targeted therapy, they were alimemazine, MG-262, fluoxetine, quipazine, naltrexone and oxybenzone. In conclusion, our current study was constructed an AML prognostic signature based on the 14 prognostic snoRNAs, which may serve as a novel prognostic biomarker for AML.
Over the past few years, there has been a growing interest in the dynamic characteristics of viscoelastic fluids driven by the requirements of hydrodynamic engineering specifically in ensuring the safety of fluid transportation in tankers, aerospace and shipbuilding industries. This interest stems not only from practical needs but also from the mathematical analysis of the associated problem.
The investigation of a system comprising a rigid body containing an ideal or viscous liquid using functional analysis methods has been the focus of several studies which can be found in fundamental monographs [1,2,3]. Furthermore, certain cases of an ordinary viscous fluid (homogeneous or heterogeneous) partially or fully filling a fixed container have been studied and discussed in [4,5,6,7].
To date, researchers have developed various analytical and numerical approaches to investigate the behavior of different structures interacting with a viscoelastic fluid [8]. Simultaneously, the study of hydrodynamic problems related to small motions and normal oscillations of viscoelastic fluids in immovable containers has extensively utilized methods based on functional analysis and the theory of operators in abstract Hilbert spaces (e.g., [9,10,11,12,13,14,15]). Recently, the specific case of a pendulum fully filled with a viscoelastic fluid was examined in [16]. Furthermore, the case of two nonmixing fluids, with the lower one being viscoelastic and the upper one inviscid was investigated in [15]. On the other hand, piezoelectric materials are introduced to examine mechanical behaviors on the stability of pendulum in presence of an electric field. To describe this effect, simultaneous coupling equations with tensor parameters associated to mechanical behaviors and the electric field are used [17,18] (and references therein).
The objective of this study is to expand upon previous works [2,16,19] by investigating the more challenging case of a pendulum that is partially filled with a viscoelastic fluid. The investigation of a pendulum containing a viscoelastic fluid topped with a barotropic gas will be reserved for another work.
Once the linearized equations of system motion (pendulum-liquid) are derived, presupposing the liquid inside the cavity to be following the simpler Oldroyd's model for viscoelastic fluids we reformulate these equations as a variational problem and then as an operatorial problem involving bounded linear operators on an appropriate Hilbert space.
Finally, through the examination of the eigenvalues of the linear operator that describes the dynamics of the coupled system we demonstrate that under the aforementioned hypotheses the given equilibrium is stable, as all eigenvalues have a non-negative real part. Moreover, if the viscosity coefficient reaches a certain threshold, the spectrum exhibits three branches of eigenvalues with potential cluster points at 0, β, and ∞. Among these eigenvalues, λ=0 possesses infinite multiplicity, while λ=β is not an eigenvalue. These three branches of eigenvalues correspond to frequencies associated with various types of waves.
It is argued that the presence of viscoelastic forces gives rise to novel physical effects that are not typically observed in an ordinary viscous incompressible fluid [2,19]. These effects are associated with the emergence of a distinct essential spectrum. Specifically, the existence of internal dissipative waves, whose decay rates are influenced by the viscoelastic parameter β of the fluid is observed.
The studied system involves a viscoelastic fluid in which the tensor of viscous stresses σ′ and the doubled tensor of deformation velocities τ satisfy a specific differential equation [2]
(1+ηddt)σ′=(κ0+κ1ddt)τ. | (2.1) |
Setting
κ0−κ1λ1−ηλ=μ(1+αγ−λ) | (2.2) |
with
γ=η−1;α=κ0κ1−γ;μ=κ1γ |
where μ is the viscosity coefficient of the solvent, γ is the inverse of the relaxation time and k0 and k1 are the total viscosity and elastic modulus of the viscoelastic fluid, respectively.
In the following, we set
I0(λ)=1+αγ−λ=β−λγ−λ;β=α+γ. | (2.3) |
It is supposed [2] that if the tensor of deformation velocity is equal to zero at the instant t=0 then the same condition holds for the tensor of viscous stresses. Under this hypothesis the differential equation (2.1) can be replaced by an integral relation
σ′=μˆI0(t)τ. | (2.4) |
Indeed, if Σ′(p) and T(p) denote the Laplace transforms of σ′ and τ, respectevely, we have
Σ′(p)=μ(1+αγ+p)T(p). | (2.5) |
From the equation (2.5), noting that 1γ+pT(p) is a product of transformed we deduce
ˆI0(t)τ(t)=τ(t)+α∫t0e−γ(t−s)τ(s)ds. | (2.6) |
The pendulum under consideration consists of a rigid rod, denoted as O0A which is firmly attached at a point A to a rigid body known as O0. The rod is constrained to rotate around a fixed point O0 referred to as the suspension point.
The body (S0) is assumed to be two-dimensional and symmetric with respect to the axis that contains O0A and with center of mass G on the rod O0A (O0G=a). Within this body (S0) there exists a symmetric cavity that is partially filled with a homogeneous incompressible viscoelastic fluid. The constitutive equation of the fluid is described by the simpler Oldroyd model [2].
In the lowest equilibrium position, Ω, S, Γ are respectively the domain occupied by the fluid, the wetted wall of the cavity and the horizontal free line [Figure 1]. Ωt, St, Γt are their positions at the instant t, respectively. We call h the distance of O0 to Γ, θ the small angle of rotation of the pendulum.
We use the fixed orthogonal axes O0x1x2 (O0x2) directed vertically upwards and orthogonal axes O′x′1x′2 fixed to the pendulum, with (O′x′1) carrying out the position of Γ at the instant t are used [Figure 1]. The acceleration →g of the gravity field is such that →g=−g→x2.
The small oscillations of the system "pendulum - viscoelastic fluid" in linear theory are going to be studied.
Let →u(x1,x2,t) be the small displacement of a fluid particle M with respect to the fixed axes O0x1x2 and →U(x1,x2,t) its displacement with respect to the mobile axes O′x′1x′2. Then,
→u=θ→z×→O0M+→U(→z=→x1×→x2). | (4.1) |
If P(x1,x2,t) is the pressure then
ρ¨ui=−∂P∂xi+∂σ′ij∂xj−ρgδi2inΩ(i,j=1,2) | (4.2) |
div→u=0incompressibility inΩ | (4.3) |
→U|S=0condition on the wallS | (4.4) |
If we denote by
τij=∂˙ui∂xj+∂˙uj∂xi(i,j=1,2) | (4.5) |
the components of the tensor τ, we have for the tensor σ′
σ′ij=μˆI0τij. | (4.6) |
Then,
∂σ′ij∂xj=μˆI0∂τij∂xj=μˆI0(∂2˙ui∂xj∂xj+∂∂xi(∂˙uj∂xj))=μˆI0Δ˙ui | (4.7) |
The equation (4.2) takes the form, since Δ→u=Δ→U:
ρ¨→U=−→gradP+μˆI0Δ˙→U−ρg→x2−¨θ→z×→O0M | (4.8) |
If it is supposed that the pressure is equal to zero above the free line, the pressure in the equilibrium position is
Pst=−ρg(x2+h). | (4.9) |
Introducing the dynamic pressure p=P−Pst, we obtain
ρ¨→U=−→gradp+μˆI0Δ˙→U−¨θ→z×→OM | (4.10) |
and the equation (4.3) can be written
div→U=0 | (4.11) |
We must add the dynamic conditions on the free line Γt whose equation is
x′2=Un|Γ | (4.12) |
or
x2=−h+θx1+Un|Γ. | (4.13) |
We have
σijnj=0onΓt | (4.14) |
or
(−Pδij+2μˆI0εij)nj=0onΓt | (4.15) |
where εij(i,j=1,2) is the rate of deformation tensor.
Or, in linear theory
([ρg(θx1+Un|Γ)−p]δij+2μˆI0εij)nj=0onΓ | (4.16) |
and finally
ˆI0ε12|Γ=0 | (4.17) |
p|Γ=ρg(θx1+Un|Γ)+2μˆI0ε22|ΓonΓ | (4.18) |
The equations (4.10), (4.11), (4.4), (4.17), (4.18) are the equations of motion of the fluid.
Let →˜U a sufficiently smooth function defined in Ω and verifying div→˜U=0 and →˜U|S=0. We have
∫Ωρ¨→U⋅ˉ→˜UdΩ=−∫Ω→gradp⋅ˉ→˜UdΩ+μˆI0∫ΩΔ˙→U⋅ˉ→˜UdΩ−ρ¨θ∫Ω(x1ˉ˜U2−x2ˉ˜U1)dΩ | (4.19) |
The Green's formula and (4.11) (4.4) give
−∫Ω→gradp⋅ˉ→˜UdΩ=−∫Ωdiv(pˉ→˜U)dΩ=−∫Γp|Γˉ˜Un|ΓdΓ | (4.20) |
The vectorial Laplacian formula [1], and (4.4), (4.17) give
ˆI0∫ΩΔ˙→U⋅ˉ→˜UdΩ=−2∫ΩˆI0ϵij(˙→U)ϵij(ˉ→˜U)dΩ+2∫ΓˆI0ϵ22|Γˉ˜Un|ΓdΓ. | (4.21) |
Therefore, we obtain
{∫Ωρ¨→U⋅ˉ→˜UdΩ=∫Γ(−p|Γ+2μˆI0ϵ22|Γ)ˉ˜Un|ΓdΓ−2∫ΩˆI0ϵij(˙→U)ϵij(ˉ→˜U)dΩ−ρ¨θ∫Ω(x1ˉ˜U2−x2ˉ˜U1)dΩ | (4.22) |
and using the dynamic condition (4.18)
{∫Ωρ¨→U⋅ˉ→˜UdΩ+2∫ΩˆI0ϵij(˙→U)ϵij(ˉ→˜U)dΩ+ρg∫Γ(θx1+Un|Γ)ˉ˜Un|ΓdΓ+ρ¨θ∫Ω(x1ˉ˜U2−x2ˉ˜U1)dΩ=0for all admissible→˜U. | (4.23) |
The assumption is going to be made that →U and →˜U belong to the space
Vdef=J10,S(Ω)def={→U∈χ1(Ω)def=[H1(Ω)]2;div→U=0inΩ;→U|S=0} | (4.24) |
equipped with the scalar product
(→U,→˜U)V=2∫Ωϵij(→U)ϵij(ˉ→˜U)dΩ; | (4.25) |
(it is well-known that the associated norm is equivalent in J10,S(Ω) to the classical norm of χ1(Ω) [1]).
We introduce the space
χdef=J0,S(Ω)def={→U∈L2(Ω)def=[L2(Ω)]2;div→U=0;Un|S=0}, | (4.26) |
equipped with the scalar product (of L2(Ω)) :
(→U,→˜U)χ=∫Ω→U⋅→˜UdΩ. | (4.27) |
It is well-known [1] that the embedding from V⊂χ is continuous, dense and compact. We denote by A the unbounded operator of χ associated to the pair (V,χ) and to the scalar product of (⋅,⋅)V.
In this section, a few operators are introduced.
We set
∫Ω(x1U2−x2U1)dΩ=L→U | (4.28) |
L being a compact operator from χ into C.
We have
¨θ∫Ω(x1ˉ˜U2−x2ˉ˜U1)dΩ=(¨θ,L→˜U)C=(L∗¨θ,→˜U)χ | (4.29) |
The adjoint L∗ is compact from C into χ.
Using a trace theorem in χ1(Ω), we can set
∫ΓUn|Γˉ˜Un|ΓdΓ=(K→U,→˜U)V, | (4.30) |
where K is not negative, selfadjoint, bounded operator from V into V.
Let us prove that K is compact.
Indeed, let {→Up} a weakly convergent sequence in V. By a trace theorem, the sequence {Upn|Γ} is strongly convergent in ˜L2(Γ)={f∈L2(Γ),∫ΓfdΓ=0} and we have
(K(→Up−→Uq),→Up−→Uq)V=∫Γ|Upn|Γ−Uqn|Γ|2dΓ→0whenp,q→∞; | (4.31) |
consequently [20], K is compact.
Let
∫Γx1Un|ΓdΓ=K1→U | (4.32) |
K1 being compact from V into C.
Then,
θ∫Γx1ˉ˜Un|ΓdΓ=(θ,K1→˜U)C=(K∗1θ,→˜U)V, | (4.33) |
where the adjoint K∗1 is compact from C into V.
Consequently, the variational equation (4.23) can be written
{ρ(¨→U,→˜U)χ+μ(ˆI0˙→U,→˜U)V+ρ(L∗¨θ,→˜U)χ+ρg(K→U,→˜U)V+ρg(K∗1θ,→˜U)V=0 | (4.34) |
for all →˜U∈V and then by density for all →˜U∈χ.
It is well-known [21] that the equation (4.34) is equivalent to the operatorial equation
¨→U+L∗¨θ+A[νˆI0˙→U+gK∗1θ+gK→U]=0,→U∈V | (4.35) |
where ν=μρ−1.
An equation with bounded coefficients is obtained by setting
A1/2→U=→W∈χ | (4.36) |
and by applying the operator A−1/2. The equation can be expressed in operator form as:
A−1¨→W+A−1/2L∗¨θ+νˆI0˙→W+gA1/2KA−1/2→W+gA1/2K∗1θ=0 | (4.37) |
Let mp and Jp be the mass and the moment of inertia about O0 of the body S0+O0A. We have the equation
Jp¨θ→z+∫Ω→O0M×ρ¨→udΩ=→O0G×(−mpg→x2)+∫Ωt→O0M×(−ρg→x2)dΩt | (5.1) |
At first, we have
∫Ω→O0M×ρ¨→udΩ=[ρ∫Ω(x1¨U2−x2¨U1)dΩ+¨θ∫Ωρ(x21+x22)dΩ]→z=[ρL¨→U+Jℓ¨θ]→z, | (5.2) |
where Jℓ is the moment of inertia about O0 of the liquid solidified in the equilibrium position.
We have
→O0G×(−mpg→x2)=−mpgaθ→z | (5.3) |
Finally, we write
∫Ωt→O0M×(−ρg→x2)dΩt=−ρg∫Ωtx1dΩt⋅→z | (5.4) |
But, we have
−ρg∫Ωtx1dΩt=−ρg∫Ωt(x′1−θx′2+hθ)dΩt | (5.5) |
=−ρg∫Ωtx′1dΩt−gθ∫Ωρ(h−x′2)dΩ | (5.6) |
We can write
∫Ωtx′1dΩt=∫Ωx′1dΩ−∫Ωt−Ωx′1dτ=∫Γx1Un|ΓdΓ, |
so that we have
−ρg∫Ωtx1dΩt=−ρg∫Γx1Un|ΓdΓ−mℓg(h−b)θ, | (5.7) |
where b is the ordinate of the center of inertia of the liquid in the equilibrium position with respect to the axes O′x′1x′2 and mℓ the mass of the liquid.
Finally, setting
m=mp+mℓ;mpa+mℓ(h−b)=mc;J=Jp+Jℓ | (5.8) |
we obtain the equation
ρL¨→U+J¨θ+ρg∫Γx1Un|ΓdΓ+mcgθ=0 | (5.9) |
or
ρL¨→U+J¨θ+ρgK1→U+mcgθ=0 | (5.10) |
or
ρLA−1/2¨→W+J¨θ+ρgK1A−1/2→W+mcgθ=0 | (5.11) |
The equations (4.37) and (5.11) are the operatorial equations of the motion of the system pendulum - fluid.
Setting J1=Jρ and m0=mcρ, the equations (4.37), (5.11) become
{A−1¨→W+A−1/2L∗¨θ+νˆI0˙→W+gA1/2KA−1/2→W+gA1/2K∗1θ=0LA−1/2¨→W+J1¨θ+gK1A−1/2→W+m0gθ=0 | (6.1) |
Using the definition of ˆI0, we have
ˆI0˙→W=˙→W(t)+α∫t0e−γ(t−s)∂→W(s)∂sds | (6.2) |
Integrating by parts, we obtain
ˆI0˙→W=˙→W(t)+α→W(t)−αγ∫t0e−γ(t−s)→W(s)ds | (6.3) |
and, setting
→W1(t)=(να)1/2∫t0e−γ(t−s)→W(s)ds, | (6.4) |
we have
νˆI0˙→W=ν˙→W(t)+να→W(t)−γ(να)1/2→W1(t) | (6.5) |
On the other hand, derivating gives us
˙→W1=−γ→W1+(να)1/2→W | (6.6) |
Finally, three equations with constant coefficients are obtained for →W, θ, →W1 (5.11), (6.6) and (6.7), where
A−1¨→W+A−1/2L∗¨θ+ν˙→W+να→W−γ(να)1/2→W1+gA1/2KA−1/2→W+gA1/2K∗1θ=0 | (6.7) |
The solutions of the precedent equations (5.11), (6.6) and (6.7) are sought depending on the time t by the law e−λt,λ∈C.
We obtain
{λ2(A−1→W+A−1/2L∗θ)−νλ→W+(ναIχ+gA1/2KA−1/2)→W−γ(να)1/2→W1+gA1/2K∗1θ=0 | (6.8) |
λ2(LA−1/2→W+J1θ)+gK1A−1/2→W+m0gθ=0 | (6.9) |
−λ→W1=−γ→W1+(να)1/2→W | (6.10) |
It should be noted that the spectrum σ(A) of linear self-adjoint operator A in Hilbert space H is divided into two parts, the discrete and the essential spectrum [1]:
a) The discrete spectrum, denoted σd(A) contains the isolated eigenvalues with finite multiplicity.
b) The essential spectrum, denoted σe(A) contains the set of points of σ(A) which are not isolated eigenvalues with finite multiplicity. Then, σe(A) is formed by the eigenvalues with infinite multiplicity, the accumulation points of eigenvalues and "continuous spectrum".
We conclude that:
σ(A)=σe(A)∪σd(A);σe(A)∩σd(A)=∅ | (6.11) |
From a physical perspective, we observe that in the oscillations of a liquid that partially fills a container the normal oscillations can be categorized into two distinct classes surface waves and internal waves:
c) The surface waves are caused by the presence of the free surface of the liquid and the gravitation, capillar, and centrifugal forces (and others...) acting on the system.
d) The internal waves whose modes have the following property: For them, the free surface of the fluid is almost unperturbed during the process of oscillations and the proper movements take place mainly inside the region fluid.
Let us recall that in spectral analysis we seek the properties of normal oscillations and their corresponding waves (surface or internal). Then, we seek λ∈C solution of the spectral problem i.e., λ∈σ(A) and the characteristics of the corresponding associated waves.
Setting λ=0 and eliminating θ and →W1, we obtain
(m0A1/2KA−1/2−A1/2K∗1K1A−1/2)→W=0 | (6.12) |
and consequently
m0(K→U,→U)V−(K1→U,K1→U)V=0 | (6.13) |
or
m0‖Un|Γ‖2˜L2(Γ)−|∫Γx1Un|ΓdΓ|2=0 | (6.14) |
Using the Schwarz inequality, we have
m0‖Un|Γ‖2˜L2(Γ)−|∫Γx1Un|ΓdΓ|2≥(m0−∫Γx21dΓ)‖Un|Γ‖2˜L2(Γ) | (6.15) |
and the right-hand side is positive if the pendulum is preponderant.
Therefore,
Un|Γ=0 | (6.16) |
or if γΓ is the normal trace of χ1(Ω) on Γ :
γΓA−1/2→W=0 | (6.17) |
i.e
→W∈KerγΓA−1/2 | (6.18) |
From Un|Γ=0, we deduce K1→U=0 or θ=0. Finally, we have
→W1=(να)1/2γ→W∈KerγΓA−1/2 | (6.19) |
The eigenspace of λ=0 is the subspace of χ⊕C defined by →W∈KerγΓA−1/2, θ=0.
If λ=γ then we have →W=0 and →W1=0. Thus
(γ2J1+m0g)θ=0orθ=0. | (6.20) |
We conclude that all proper oscillations of the considered hydrodynamic system cannot be represented with fading decrements e(−γt). On the other hand, we can divide by the number (λ−γ≠0). Then, the expression I0(λ)=λ−βλ−γ is true.
Consequently, for studying the spectrum, we can use the equation (6.10) and we obtain only two equations for →W, θ.
Setting
I0(λ)=λ−βλ−γ | (6.21) |
we have
λ2(A−1→W+A−1/2L∗θ)+(−νλI0(λ)Iχ+gA1/2KA−1/2)→W+gA1/2K∗1θ=0 | (6.22) |
(λ2LA−1/2+gK1A−1/2)→W+(λ2J1+m0g)θ=0 | (6.23) |
The case →W=→0 or →U=→0 is dismissed, i.e the case where the liquid is solidified in the equilibrium position. Then, we have the classical pendulum whose pulsation ω is given by ω2=m0gJ1.
Eliminating θ, we have the unique equation for →W :
{λ2A−1→W−(λ2A−1/2L∗+gA1/2K∗1)(λ2LA−1/2+gK1A−1/2)(λ2J1+m0g)→W+(−νλI0(λ)Iχ+gA1/2KA−1/2)→W=0 | (6.24) |
Dividing the equation (4.22) by −νλI0(λ), we obtain
{[Iχ−λA−1νI0(λ)+(λ2A−1/2L∗+gA1/2K∗1)(λ2LA−1/2+gK1A−1/2)νλI0(λ)(λ2J1+m0g)−gA1/2KA−1/2νλI0(λ)]→W=0 | (6.25) |
The terms between the brackets are selfadjoint and are compact, except Iχ.
Therefore, we have a Fredholm pencil in the domain
C−{0}−{β}−{±i√m0gJ1}, |
regular in this domain since λ=γ is not an eigenvalue [1].
Consequently, the spectrum of the problem exists; it is formed by isolate points and its possible points of accumulation can be only 0,β,±i√m0gJ1and∞.
On the other hand, if the pencil is selfadjoint, the spectrum is symmetrical with respect to the real axis.
1) Stability of equilibrium : Discarding the eigenvalue λ=0, we divide by λ the equation (6.22) and (6.23).
We obtain
(λQ+1λB−(νI0(λ)Iχ000))Z=0 | (6.26) |
with
Z=(→Wθ)∈χ⊕C |
and
Q=(A−1A−1/2L∗LA−1/2J1IC);B=g(A1/2KA−1/2A1/2K∗1K1A−1/2m0IC) |
We have
(QZ,Z)χ⊕C=(A−1→W,→W)χ⊕C+2ℜ(LA−1/2→W,θ)C+J1|θ|2=‖→U‖2χ+2ℜ(L→U,θ)C+J1|θ|2 | (6.27) |
Since
|(L→U,θ)C|=|∫Ω(x1U2−x2U1)dΩ⋅ˉθ|≤√Jℓρ‖→U‖χ|θ|, | (6.28) |
we have
(QZ,Z)χ⊕C≥‖→U‖2χ−2√Jℓρ‖→U‖χ|θ|+J1|θ|2, | (6.29) |
so that, since J1>Jℓρ−1, (QZ,Z) is definite positive quadratic form of ‖→U‖χ and |θ|, then equal to zero only for Z=0.
Now, we have, in the same manner
(BZ,Z)χ⊕C≥g[‖Un|Γ‖2˜L2(Γ)−2√∫Γx21dΓ‖Un|Γ‖˜L2(Γ)|θ|+m0|θ|2], | (6.30) |
so that, since m0−∫Γx21dΓ>0, we have
(BZ,Z)≥0,equal to zero forUn|Γ=0,θ=0. | (6.31) |
From the equation (6.26), we deduce
λ(QZ,Z)+1λ(BZ,Z)=νI0(λ)‖→W‖2χ | (6.32) |
Taking the real parts, we obtain
ℜλ[(QZ,Z)+1|λ|2(BZ,Z)]=νℜI0(λ)‖→W‖2χ | (6.33) |
or
ℜλ[(QZ,Z)+1|λ|2(BZ,Z)+νβ−γ|λ−γ|2‖→W‖2χ]=ν|λ|2+βγ|λ−γ|2‖→W‖2χ | (6.34) |
so
ℜλ>0 | (6.35) |
and the equilibrium of the system is stable in linear approximation.
2) Location of the eigenvalues :
From (6.34), we deduce ℜI0(λ)>0 or
|λ|2+βγ−(β+γ)ℜλ>0 | (6.36) |
Setting, λ=x+iy, we obtain
(x−β+γ2)+y2>α24. | (6.37) |
The eigenvalues are in the not dotted part of the plane (λ).
If λ=β is an eigenvalue then there exists →W≠0 such that
{β2(A−1→W,→W)χ+g(A1/2KA−1/2→W,→W)χ−((β2A−1/2L∗+gA1/2K∗1)(β2LA−1/2+gK1A−1/2)(β2J1+m0g)→W,→W)χ=0 | (6.38) |
This equality can be written
{(β2J1+m0g)[β2‖→U‖2χ+g‖Un|Γ‖2˜L2(Γ)]−|β2∫Ω(x1U2−x2U1)dΩ+g∫Γx1Un|ΓdΓ|2=0 | (6.39) |
Using the Cauchy's inequality for the second term, we have
{[β2m0g+β4(J1−2Jℓρ)]‖→U‖2χ+[β2gJ1+g2(m0−2∫Γx21dΓ)]‖Un|Γ‖2˜L2(Γ)≤0 | (6.40) |
If the pendulum is preponderant
J1−2Jℓρ=Jp−Jℓρ>0;m0−2∫Γx21dΓ>0, | (6.41) |
the left-hand side is equal to zero only for →U=0 then for →W=0.
Therefore λ=β is not an eigenvalue and we can note that all proper oscillations of the considered hydrodynamic system can not be represented with fading decrements e(−βt). On the other hand, we can divide by (λ−β≠0) then (I0(λ))−1=λ−γλ−β exist and we can replace the pencil L(λ) by the pencil Ł0(λ)=−1νI0(λ)L(λ) (see section 7.2).
We consider the pencil L(λ) defined by :
L(λ)→W={λ2A−1+(−νλI0(λ)Iχ+gA1/2KA−1/2)−(λ2A−1/2L∗+gA1/2K∗1)(λ2LA−1/2+gK1A−1/2)(λ2J1+m0g)}→W=0 | (7.1) |
Setting
λ′=λ−1,ˆL(λ′)=λ′2L(1λ′), | (7.2) |
we obtain
{ˆL(λ′)=A−1−(A−1/2L∗+λ′2gA1/2K∗1)(LA−1/2+λ′2gK1A−1/2)(J1+m0gλ′2)+(−νλ′(1−βλ′)1−γλ′Iχ+gA1/2KA−1/2) | (7.3) |
ˆL(λ′) is a self adjoint operatorial function that is holomorphic in the vicinity of λ′=0.
We have
{ˆL(0)=A−1−A−1/2L∗LA−1/2J1+gA1/2KA−1/2compactˆL′(0)=−νβγIχstrongly negative | (7.4) |
Let us prove that KerˆL(0)=0.
We have
(ˆL(0)→W,→W)χ≥g‖Un|Γ‖2˜L2(Γ)+JpJ‖→U‖2χ, | (7.5) |
ˆL(0)→W=0 only for →U=0 or →W=0.
Consequently [1], for each ε>0 sufficiently small in ]0,ε[ there exists an infinity of positive real eigenvalues λ′n having zero as point of accumulation. For our problem, there exists an infinity of positive real eigenvalues λn=λ′−1n having the infinity as point of accumulation. The corresponding eigenelements form a Riesz basis in a subspace of χ having a finite defect.
Dividing L(λ) by −νλI0(λ), we obtain the pencil
{Ł0(λ)=Iχ−ν−1λ−γλ(λ−β)gA1/2KA−1/2−ν−1λ(λ−γ)λ−βA−1−ν−1λ−γλ(λ−β)(λ2A−1/2L∗+gA1/2K∗1)(λ2LA−1/2+gK1A−1/2)(λ2J1+m0g) | (7.6) |
After calculations, we have
λ(λ−γ)λ−β=λ+α+O(1λ) | (7.7) |
and for the last term
ν−1J1(λ+α)A−1/2L∗LA−1/2+O(1λ) |
so that we can write
{Ł0(λ)=Iχ−ν−1α(A−1+J−11A1/2LL∗A−1/2)−λν−1(A−1+J−1A1/2LL∗A−1/2)+O(1λ) | (7.8) |
But, we have the following theorem [1] :
Let the pencil
Ł0(λ)=I+T−λQ+B(λ) | (7.9) |
with T compact, Q selfadjoint, positive definite, compact, B(λ) analytic outside the circle |λ|≤R and turning into zero at infinity.
We have
λn(Ł0(λ))=1λn(Q)[1+o(1)],n⟶∞ | (7.10) |
Here, the conditions are satisfied and we have
λn=νλn(A−1+J−11A1/2LL∗A−1/2)[1+o(1)],n⟶∞ | (7.11) |
Let us consider still the pencil L(λ).
L(λ) is a self adjoint operatorial function, holomorphic in the vicinity of λ=0.
We have
{L(0)=−gm0A1/2K∗1K1A−1/2+gA1/2KA−1/2compactL′(0)=−νβγIχstrictly negative | (8.1) |
On the other hand, L(0) admits λ=0 as eigenvalue with infinite multiplicity because we have
(m0A1/2KA−1/2−A1/2K∗1K1A−1/2)→W=0if→W∈KerγΓA−1/2. | (8.2) |
Therefore, for all ε>0 sufficiently small there exists in ]0,ε[ an infinity of positive real eigenvalues λ0n having zero as point of accumulation. The corresponding eigenelements and an orthonormal basis of KerγΓA−1/2 form a Riesz basis in a subspace of χ having a finite defect.
Setting
λ=λ"+β,˜L(λ")=L(λ"+β), | (9.1) |
we have
˜L(λ")→W={(λ"+β)2A−1+(−νλ"λ"+βλ"+αIχ+gA1/2KA−1/2)−[(λ"+β)2A−1/2L∗+gA1/2K∗1][(λ"+β)2LA−1/2+gK1A−1/2]((λ"+β)2J1+m0g)}→W=0 | (9.2) |
Let
F(ψ)=(ψA−1/2L∗+gA1/2K∗1)(ψLA−1/2+gK1A−1/2)(ψJ1+m0g) |
For each ψ, this operator is compact.
We have
{˜L(0)=β2A−1−F(β2)+gA1/2KA−1/2compact˜L′(0)=2βA−1−2βF′(β2)−νβαIχ | (9.3) |
with A−1 and F′(β2) being bounded operators, ˜L′(0) is strongly negative if ν is sufficiently large.
On the other hand, λ"=0 cannot be an eigenvalue of ˜L(λ") since β is not an eigenvalue of L(λ). Therefore, if the viscosity coefficient is sufficiently large and if the pendulum is preponderant, for each ε>0 sufficiently small, there is in ]0,ε[ an infinity of positive real eigenvalues λ″n having zero as point of accumulation. So for our problem, in ]β,β+ε[ there is an infinity of real eigenvalues λβn=λ″n+β having λ=β as accumulation point. The eigenelements form a Riesz basis in a subspace of χ having a finite defect.
This study builds upon previous works [2,16,19] that investigated the normal oscillations of a pendulum with a cavity partially or fully filled with fluid. Specifically, in [2,19] the model of a cavity partially filled with an ordinary viscous fluid was examined. In [16], a special case was explored where the cavity is completely filled with a viscoelastic fluid. It was demonstrated that the presence of viscoelastic forces leads to a more complex structure of normal oscillation waves notably resulting in the emergence of internal dissipative waves within the fluid region.
Let us state some important results and explain their physical meaning.
1) If the pendulum is preponderant, it is proved that the lowest equilibrium position of the considered hydrodynamic system is stable in linear approximation.
2) For a large viscosity of the viscoelastic fluid, the spectrum consisting of three branches of real eigenvalues (λ0n)n, (λ∞n)n and (λβn)n with limiting points 0,∞ and β, and corresponding to various kinds of normal oscillation waves. If we take θ=0, we have the same results for normal oscillations of a viscoelastic fluid in an open container [2] (section 11.3) and [11].
3) It is proved that the new branch (λβn) of finite multiplicity eigenvalues, that corresponds on the viscoelastic parameter β=κ0κ1 of the fluid satisfied : λβn→β (n→+∞). This result confirms the fundamental influence of the viscoelastic forces on the structure of the spectrum of the considered hydrodynamic system. Physically, wave motions corresponding to these eigenvalues are predominantly internal in nature and arise exclusively from the action of viscoelastic forces. We remark that this effect disappears in the case of an ordinary viscous fluid [2,19], where the elastic parameter α=0, then β=γ and I0(λ)=1. This gives that the viscoelastic forces in fluids have a significant impact onto the asymptotic distribution of the eigenvalues related to the internal waves inside the region fluid.
4) For the limit points λ=∞, we have the branch (λ∞n)n of finite multiplicity eigenvalues that corresponds to the internal dissipative waves in the viscoelastic fluid and just as in the case of an ordinary viscous fluid [2,19]. For these waves, the fading decrements can be as large as possible.
5) For the limit points λ=0, we have the branch (λ0n)n of finite multiplicity eigenvalues that corresponds to the surface waves with decrements of the oscillation fading as small as possible. Their appearance is caused by the presence of the free surface of the fluid and by the influence of the gravitation field. This type of wave motions in fluids disappears (in particular λ=0 is not an eigenvalue) in [16] where the cavity is completely filled by a viscoelastic fluid.
6) When the effects of elastic forces inside the viscoelastic fluid are absent the elastic parameter α=0, β=γ and I0(λ)=1. Therefore, the problem (4.24) is reduced to the study of a classical Askerov, Krein, Laptev pencil for studying small motions of viscous fluid in arbitrary open container. The small motions of the system depend on the viscosity coefficient of the fluid, and we have the well known results for a pendulum partially filled with an ordinary viscous liquid [2,19].
i) For a large viscosity coefficient there is a set of real eigenvalues λ+n→∞ corresponding to arbitrary strongly damped aperiodic motions (internal dissipative waves) and a set of real eigenvalues λ−n→0, corresponding to arbitrary weakly damped aperiodic motions (surface waves).
ii) For a small viscosity coefficient, there is a finite number of complex eigenvalues corresponding to damped "oscillatory" motions.
In conclusion, we can confirm that this problem is new both in its hydrodynamic statement and as a problem in mathematical physics. It is shown that the visco-elastic forces are the cause of new physical effects that are not characteristic for an ordinary viscous incompressible fluid filling completely or partially a certain moving vessels. We believe that problems of this kind will capture the attention of other researchers. These are two important directions in which we believe that the studies should directed in the future.
1) For pendulum, piezoelectric materials can be used to examine mechanical behaviors on the stability of pendulum with viscoelastic fluid in presence of an electric field.
2) For fluid inside the cavity, relaxation property can be introduced, and dynamical characteristics of pendulum can be investigated in relation with this new physical fluid property.
The authors declare they have not used Artificial Intelligence (AI) tools in the creation of this article.
The authors are grateful to the referees and the editorial board for some useful comments that improved the presentation of the manuscript.
The authors do not have any competing interests in the manuscript.
[1] |
A. Khwaja, M. Bjorkholm, R. E. Gale, R. L. Levine, C. T. Jordan, G. Ehninger, et al., Acute myeloid leukaemia, Nat. Rev. Dis. Primers, 2 (2016), 16010. https://doi.org/10.1038/nrdp.2016.10 doi: 10.1038/nrdp.2016.10
![]() |
[2] |
E. Estey, H. Dohner, Acute myeloid leukaemia, Lancet, 368 (2006), 1894-1907. https://doi.org/10.1016/S0140-6736(06)69780-8 doi: 10.1016/S0140-6736(06)69780-8
![]() |
[3] |
L. Bullinger, K. Dohner, E. Bair, S. Frohling, R. F. Schlenk, R. Tibshirani, et al., Use of gene-expression profiling to identify prognostic subclasses in adult acute myeloid leukemia, N. Eng. J. Med., 350 (2004), 1605-1616. https://doi.org/10.1056/NEJMoa031046 doi: 10.1056/NEJMoa031046
![]() |
[4] |
E. Papaemmanuil, M. Gerstung, L. Bullinger, V. I. Gaidzik, P. Paschka, N. D. Roberts, et al., Genomic classification and prognosis in acute myeloid leukemia, N. Eng. J. Med., 374 (2016), 2209-2221. https://doi.org/10.1056/NEJMoa1516192 doi: 10.1056/NEJMoa1516192
![]() |
[5] |
C. C. Coombs, M. S. Tallman, R. L. Levine, Molecular therapy for acute myeloid leukaemia, Nat. Rev. Clin. Oncol., 13 (2016), 305-318. https://doi.org/10.1038/nrclinonc.2015.210 doi: 10.1038/nrclinonc.2015.210
![]() |
[6] |
J. W. Tyner, C. E. Tognon, D. Bottomly, B. Wilmot, S. E. Kurtz, S. L. Savage, et al., Functional genomic landscape of acute myeloid leukaemia, Nature, 562 (2018), 526-531. https://doi.org/10.1038/s41586-018-0623-z doi: 10.1038/s41586-018-0623-z
![]() |
[7] |
S. Abelson, G. Collord, S. W. K. Ng, O. Weissbrod, N. M. Cohen, E. Niemeyer, et al., Prediction of acute myeloid leukaemia risk in healthy individuals, Nature, 559 (2018), 400-404. https://doi.org/10.1038/s41586-018-0317-6 doi: 10.1038/s41586-018-0317-6
![]() |
[8] |
S. C. Meyer, R. L. Levine, Translational implications of somatic genomics in acute myeloid leukaemia, Lancet Oncol., 15 (2014), e382-394. https://doi.org/10.1016/S1470-2045(14)70008-7 doi: 10.1016/S1470-2045(14)70008-7
![]() |
[9] |
T. Bratkovic, J. Bozic, B. Rogelj, Functional diversity of small nucleolar RNAs, Nucleic Acids Res., 48 (2020), 1627-1651. https://doi.org/10.1093/nar/gkz1140 doi: 10.1093/nar/gkz1140
![]() |
[10] |
J. Ni, A. L. Tien, M. J. Fournier, Small nucleolar RNAs direct site-specific synthesis of pseudouridine in ribosomal RNA, Cell, 89 (1997), 565-573. https://doi.org/10.1016/s0092-8674(00)80238-x doi: 10.1016/s0092-8674(00)80238-x
![]() |
[11] |
V. Chikne, K. S. Rajan, M. Shalev-Benami, K. Decker, S. Cohen-Chalamish, H. Madmoni, et al., Small nucleolar RNAs controlling rRNA processing in Trypanosoma brucei, Nucleic Acids Res., 47 (2019), 2609-2629. https://doi.org/10.1093/nar/gky1287 doi: 10.1093/nar/gky1287
![]() |
[12] |
L. Xing, X. Zhang, X. Zhang, D. Tong, Expression scoring of a small-nucleolar-RNA signature identified by machine learning serves as a prognostic predictor for head and neck cancer, J. Cell Phys., 235 (2020), 8071-8084. https://doi.org/10.1002/jcp.29462 doi: 10.1002/jcp.29462
![]() |
[13] |
Y. Zhao, Y. Yan, R. Ma, X. Lv, L. Zhang, J. Wang, et al., Expression signature of six-snoRNA serves as novel non-invasive biomarker for diagnosis and prognosis prediction of renal clear cell carcinoma, J. Cell Mol. Med., 24 (2020), 2215-2228. https://doi.org/10.1111/jcmm.14886 doi: 10.1111/jcmm.14886
![]() |
[14] | L. Huang, X. Z. Liang, Y. Deng, Y. B. Liang, X. Zhu, X. Y. Liang, et al., Prognostic value of small nucleolar RNAs (snoRNAs) for colon adenocarcinoma based on RNA sequencing data, Pathol. Res. Pract., 216 (2020), 152937. https: //doi.org/10.1016/j.prp.2020.152937 |
[15] |
J. Gong, Y. Li, C. J. Liu, Y. Xiang, C. Li, Y. Ye, et al., A pan-cancer analysis of the expression and clinical relevance of small nucleolar RNAs in human cancer, Cell Rep., 21 (2017), 1968-1981. https://doi.org/10.1016/j.celrep.2017.10.070 doi: 10.1016/j.celrep.2017.10.070
![]() |
[16] | The Cancer Genome Atlas Research Network, Genomic and epigenomic landscapes of adult de novo acute myeloid leukemia, N. Eng. J. Med., 368 (2013), 2059-2074. https://doi.org/10.1056/NEJMoa1301689 |
[17] |
M. D. Robinson, D. J. McCarthy, G. K. Smyth, edgeR: a Bioconductor package for differential expression analysis of digital gene expression data, Bioinformatics, 26 (2010), 139-140. https://doi.org/10.1093/bioinformatics/btp616 doi: 10.1093/bioinformatics/btp616
![]() |
[18] |
R. Huang, X. Liao, Q. Li, Identification and validation of potential prognostic gene biomarkers for predicting survival in patients with acute myeloid leukemia, Oncol. Targets Ther., 10 (2017), 5243-5254. https://doi.org/10.2147/OTT.S147717 doi: 10.2147/OTT.S147717
![]() |
[19] |
X. Liao, X. Wang, K. Huang, C. Yang, T. Yu, C. Han, et al., Genome-scale analysis to identify prognostic microRNA biomarkers in patients with early stage pancreatic ductal adenocarcinoma after pancreaticoduodenectomy, Cancer Manage. Res., 10 (2018), 2537-2551. https://doi.org/10.2147/CMAR.S168351 doi: 10.2147/CMAR.S168351
![]() |
[20] |
X. Liao, X. Wang, K. Huang, C. Han, J. Deng, T. Yu, et al., Integrated analysis of competing endogenous RNA network revealing potential prognostic biomarkers of hepatocellular carcinoma, J. Cancer, 10 (2019), 3267-3283. https://doi.org/10.7150/jca.29986 doi: 10.7150/jca.29986
![]() |
[21] |
W. H. Da, B. T. Sherman, R. A. Lempicki, Systematic and integrative analysis of large gene lists using DAVID bioinformatics resources, Nat. Protoc., 4 (2009), 44-57. https://doi.org/10.1038/nprot.2008.211 doi: 10.1038/nprot.2008.211
![]() |
[22] |
V. K. Mootha, C. M. Lindgren, K. F. Eriksson, A. Subramanian, S. Sihag, J. Lehar, et al., PGC-1alpha-responsive genes involved in oxidative phosphorylation are coordinately downregulated in human diabetes, Nat. Genet., 34 (2003), 267-273. https://doi.org/10.1038/ng1180 doi: 10.1038/ng1180
![]() |
[23] |
A. Subramanian, P. Tamayo, V. K. Mootha, S. Mukherjee, B. L. Ebert, M. A. Gillette, et al., Gene set enrichment analysis: a knowledge-based approach for interpreting genome-wide expression profiles, Proc. Nat. Acad. Sci. U. S. A., 102 (2005), 15545-15550. https://doi.org/10.1073/pnas.0506580102 doi: 10.1073/pnas.0506580102
![]() |
[24] |
A. Liberzon, C. Birger, H. Thorvaldsdottir, M. Ghandi, J. P. Mesirov, P. Tamayo, The molecular signatures database (MSigDB) hallmark gene set collection, Cell Syst., 1 (2015), 417-425. https://doi.org/10.1016/j.cels.2015.12.004 doi: 10.1016/j.cels.2015.12.004
![]() |
[25] |
A. Liberzon, A. Subramanian, R. Pinchback, H. Thorvaldsdottir, P. Tamayo, J. P. Mesirov, Molecular signatures database (MSigDB) 3.0, Bioinformatics, 27 (2011), 1739-1740. https://doi.org/10.1093/bioinformatics/btr260 doi: 10.1093/bioinformatics/btr260
![]() |
[26] |
J. Lamb, The connectivity map: a new tool for biomedical research, Nat. Rev. Cancer, 7 (2007), 54-60. https://doi.org/10.1038/nrc2044 doi: 10.1038/nrc2044
![]() |
[27] |
J. Lamb, E. D. Crawford, D. Peck, J. W. Modell, I. C. Blat, M. J. Wrobel, et al., The connectivity map: using gene-expression signatures to connect small molecules, genes, and disease, Science, 313 (2006), 1929-1935. https://doi.org/10.1126/science.1132939 doi: 10.1126/science.1132939
![]() |
[28] | E. W. Sayers, J. Beck, J. R. Brister, E. E. Bolton, K. Canese, D. C. Comeau, et al., Database resources of the national center for biotechnology information, Nucleic Acids Res., 48 (2020), D9-D16. https://doi.org/10.1093/nar/gkz899 |
[29] | S. Kim, J. Chen, T. Cheng, A. Gindulyte, J. He, S. He, et al., PubChem 2019 update: improved access to chemical data, Nucleic Acids Res., 47 (2019), D1102-D1109. https://doi.org/10.1093/nar/gky1033 |
[30] | M. Kuhn, D. Szklarczyk, A. Franceschini, M. Campillos, C. V. Mering, L. J. Jensen, et al., STITCH 2: an interaction network database for small molecules and proteins, Nucleic Acids Res., 38 (2010), D552-556. https://doi.org/10.1093/nar/gkp937 |
[31] | M. Kuhn, C. V. Mering, M. Campillos, L. J. Jensen, P. Bork, STITCH: interaction networks of chemicals and proteins, Nucleic Acids Res., 36 (2008), D684-688. https://doi.org/10.1093/nar/gkm795 |
[32] | D. Szklarczyk, A. Santos, C. V. Mering, L. J. Jensen, P. Bork, M. Kuhn, STITCH 5: augmenting protein-chemical interaction networks with tissue and affinity data, Nucleic Acids Res., 44 (2016), D380-384. https://doi.org/10.1093/nar/gkv1277 |
[33] | K. Yoshihara, M. Shahmoradgoli, E. Martinez, R. Vegesna, H. Kim, W. Torres-Garcia, et al., Inferring tumour purity and stromal and immune cell admixture from expression data, Nat. Commun., 4 (2013), 2612. https://doi.org/10.1038/ncomms3612 |
[34] |
B. Chen, M. S. Khodadoust, C. L. Liu, A. M. Newman, A. A. Alizadeh, Profiling tumor infiltrating immune cells with CIBERSORT, Methods Mol. Biol., 1711 (2018), 243-259. https://doi.org/10.1007/978-1-4939-7493-1_12 doi: 10.1007/978-1-4939-7493-1_12
![]() |
[35] |
Y. Benjamini, D. Drai, G. Elmer, N. Kafkafi, I. Golani, Controlling the false discovery rate in behavior genetics research, Behav. Brain Res., 125 (2001), 279-284. https://doi.org/10.1016/s0166-4328(01)00297-2 doi: 10.1016/s0166-4328(01)00297-2
![]() |
[36] |
P. Shannon, A. Markiel, O. Ozier, N. S. Baliga, J. T. Wang, D. Ramage, et al., Cytoscape: a software environment for integrated models of biomolecular interaction networks, Genome Res., 13 (2003), 2498-2504. https://doi.org/10.1101/gr.1239303 doi: 10.1101/gr.1239303
![]() |
[37] | D. Otasek, J. H. Morris, J. Boucas, A. R. Pico, B. Demchak, Cytoscape automation: empowering workflow-based network analysis, Genome Biol., 20 (2019), 185. https://doi.org/10.1186/s13059-019-1758-4 |
[38] | D. Ronchetti, L. Mosca, G. Cutrona, G. Tuana, M. Gentile, S. Fabris, et al., Small nucleolar RNAs as new biomarkers in chronic lymphocytic leukemia, BMC Med. Genomics, 6 (2013), 27. https://doi.org/10.1186/1755-8794-6-27 |
[39] |
E. Bignotti, S. Calza, R. A. Tassi, L. Zanotti, E. Bandiera, E. Sartori, et al., Identification of stably expressed reference small non-coding RNAs for microRNA quantification in high-grade serous ovarian carcinoma tissues, J. Cell Mol. Med., 20 (2016), 2341-2348. https://doi.org/10.1111/jcmm.12927 doi: 10.1111/jcmm.12927
![]() |
[40] |
L. H. Mao, S. Y. Chen, X. Q. Li, F. Xu, J. Lei, Q. L. Wang, et al., LncRNA-LALR1 upregulates small nucleolar RNA SNORD72 to promote growth and invasion of hepatocellular carcinoma, Aging (Albany NY), 12 (2020), 4527-4546. https://doi.org/10.18632/aging.102907 doi: 10.18632/aging.102907
![]() |
[41] |
F. G. Lafaille, O. Harschnitz, Y. S. Lee, P. Zhang, M. L. Hasek, G. Kerner, et al., Human SNORA31 variations impair cortical neuron-intrinsic immunity to HSV-1 and underlie herpes simplex encephalitis, Nat. Med., 25 (2019), 1873-1884. https://doi.org/10.1038/s41591-019-0672-3 doi: 10.1038/s41591-019-0672-3
![]() |
[42] |
H. Davanian, A. Balasiddaiah, R. Heymann, M. Sundstrom, P. Redenstrom, M. Silfverberg, et al., Ameloblastoma RNA profiling uncovers a distinct non-coding RNA signature, Oncotarget, 8 (2017), 4530-4542. https://doi.org/10.18632/oncotarget.13889 doi: 10.18632/oncotarget.13889
![]() |
[43] | I. Nepstad, K. J. Hatfield, I. S. Gronningsaeter, H. Reikvam, The PI3K-Akt-mTOR signaling pathway in human acute myeloid leukemia (AML) cells, Int. J. Mol. Sci., 21 (2020), 2907. https://doi.org/10.3390/ijms21082907 |
[44] |
L. Herschbein, J. L. Liesveld, Dueling for dual inhibition: Means to enhance effectiveness of PI3K/Akt/mTOR inhibitors in AML, Blood Rev., 32 (2018), 235-248. https://doi.org/10.1016/j.blre.2017.11.006 doi: 10.1016/j.blre.2017.11.006
![]() |
[45] |
J. Bertacchini, N. Heidari, L. Mediani, S. Capitani, M. Shahjahani, A. Ahmadzadeh, et al., Targeting PI3K/AKT/mTOR network for treatment of leukemia, Cell Mol. Life Sci., 72 (2015), 2337-2347. https://doi.org/10.1007/s00018-015-1867-5 doi: 10.1007/s00018-015-1867-5
![]() |
[46] |
Y. Su, X. Li, J. Ma, J. Zhao, S. Liu, G. Wang, et al., Targeting PI3K, mTOR, ERK and Bcl-2 signaling network shows superior antileukemic activity against AML ex vivo, Biochem. Pharmacol., 148 (2018), 13-26. https://doi.org/10.1016/j.bcp.2017.11.022 doi: 10.1016/j.bcp.2017.11.022
![]() |
[47] |
Y. Tabe, A. Tafuri, K. Sekihara, H. Yang, M. Konopleva, Inhibition of mTOR kinase as a therapeutic target for acute myeloid leukemia, Expert Opin. Ther. Targets, 21 (2017), 705-714. https://doi.org/10.1080/14728222.2017.1333600 doi: 10.1080/14728222.2017.1333600
![]() |
[48] |
N. Guo, M. Azadniv, M. Coppage, M. Nemer, J. Mendler, M. Becker, et al., Effects of neddylation and mTOR inhibition in acute myelogenous leukemia, Transl. Oncol., 12 (2019), 602-613. https://doi.org/10.1016/j.tranon.2019.01.001 doi: 10.1016/j.tranon.2019.01.001
![]() |
[49] |
J. Wu, G. Hu, Y. Dong, R. Ma, Z. Yu, S. Jiang, et al., Matrine induces Akt/mTOR signalling inhibition-mediated autophagy and apoptosis in acute myeloid leukaemia cells, J. Cell Mol. Med., 21 (2017), 1171-1181. https://doi.org/10.1111/jcmm.13049 doi: 10.1111/jcmm.13049
![]() |
[50] | Y. Feng, L. Wu, mTOR up-regulation of PFKFB3 is essential for acute myeloid leukemia cell survival, Biochem. Biophys. Res. Commun., 483 (2017), 897-903. |
[51] |
J. Bertacchini, C. Frasson, F. Chiarini, D. D'Avella, B. Accordi, L. Anselmi, et al., Dual inhibition of PI3K/mTOR signaling in chemoresistant AML primary cells, Adv. Biol. Regul., 68 (2018), 2-9. https://doi.org/10.1016/j.jbior.2018.03.001 doi: 10.1016/j.jbior.2018.03.001
![]() |
[52] |
V. Stavropoulou, S. Kaspar, L. Brault, M. A. Sanders, S. Juge, S. Morettini, et al., MLL-AF9 expression in hematopoietic stem cells drives a highly invasive AML expressing EMT-related genes linked to poor outcome, Cancer Cell, 30 (2016), 43-58. https://doi.org/10.1016/j.ccell.2016.05.011 doi: 10.1016/j.ccell.2016.05.011
![]() |
[53] |
T. J. Zhang, J. D. Zhou, J. C. Ma, Z. Q. Deng, Z. Qian, D. M. Yao, et al., CDH1 (E-cadherin) expression independently affects clinical outcome in acute myeloid leukemia with normal cytogenetics, Clin. Chem. Lab. Med., 55 (2017), 123-131. https://doi.org/10.1515/cclm-2016-0205 doi: 10.1515/cclm-2016-0205
![]() |
[54] | S. Wu, Y. Du, J. Beckford, H. Alachkar, Upregulation of the EMT marker vimentin is associated with poor clinical outcome in acute myeloid leukemia, J. Transl. Med., 16 (2018), 170. https://doi.org/10.1186/s12967-018-1539-y |
[55] |
L. Zhong, J. Chen, X. Huang, Y. Li, T. Jiang, Monitoring immunoglobulin heavy chain and T-cell receptor gene rearrangement in cfDNA as minimal residual disease detection for patients with acute myeloid leukemia, Oncol. Lett., 16 (2018), 2279-2288. https://doi.org/10.3892/ol.2018.8966 doi: 10.3892/ol.2018.8966
![]() |
[56] |
A. G. Chapuis, D. N. Egan, M. Bar, T. M. Schmitt, M. S. McAfee, K. G. Paulson, et al., T cell receptor gene therapy targeting WT1 prevents acute myeloid leukemia relapse post-transplant, Nat. Med., 25 (2019), 1064-1072. https://doi.org/10.1038/s41591-019-0472-9 doi: 10.1038/s41591-019-0472-9
![]() |
[57] |
H. J. Stauss, S. Thomas, M. Cesco-Gaspere, D. P. Hart, S. A. Xue, A. Holler, et al., WT1-specific T cell receptor gene therapy: improving TCR function in transduced T cells, Blood Cells Mol. Dis., 40 (2008), 113-116. https://doi.org/10.1016/j.bcmd.2007.06.018 doi: 10.1016/j.bcmd.2007.06.018
![]() |
[58] |
Y. Wang, A. V. Krivtsov, A. U. Sinha, T. E. North, W. Goessling, Z. Feng, et al., The Wnt/beta-catenin pathway is required for the development of leukemia stem cells in AML, Science, 327 (2010), 1650-1653. https://doi.org/10.1126/science.1186624 doi: 10.1126/science.1186624
![]() |
[59] | A. M. Gruszka, D. Valli, M. Alcalay, Wnt signalling in acute myeloid leukaemia, Cells, 8 (2019), 1403. https://doi.org/10.3390/cells8111403 |
[60] | F. J. Staal, F. Famili, L. G. Perez, K. Pike-Overzet, Aberrant Wnt signaling in leukemia, Cancers (Basel), 8 (2016), 78. https://doi.org/10.3390/cancers8090078 |
[61] |
A. Valencia, J. Roman-Gomez, J. Cervera, E. Such, E. Barragan, P. Bolufer, et al., Wnt signaling pathway is epigenetically regulated by methylation of Wnt antagonists in acute myeloid leukemia, Leukemia, 23 (2009), 1658-1666. https://doi.org/10.1038/leu.2009.86 doi: 10.1038/leu.2009.86
![]() |
[62] |
E. A. Griffiths, S. D. Gore, C. Hooker, M. A. McDevitt, J. E. Karp, B. D. Smith, et al., Acute myeloid leukemia is characterized by Wnt pathway inhibitor promoter hypermethylation, Leuk. Lymphoma, 51 (2010), 1711-1719. https://doi.org/10.3109/10428194.2010.496505 doi: 10.3109/10428194.2010.496505
![]() |
[63] |
C. Gasparini, C. Celeghini, L. Monasta, G. Zauli, NF-kappaB pathways in hematological malignancies, Cell Mol. Life Sci., 71 (2014), 2083-2102. https://doi.org/10.1007/s00018-013-1545-4 doi: 10.1007/s00018-013-1545-4
![]() |
[64] |
M. Breccia, G. Alimena, NF-kappaB as a potential therapeutic target in myelodysplastic syndromes and acute myeloid leukemia, Expert Opin. Ther. Targets, 14 (2010), 1157-1176. https://doi.org/10.1517/14728222.2010.522570 doi: 10.1517/14728222.2010.522570
![]() |
[65] |
M. C. Bosman, J. J. Schuringa, E. Vellenga, Constitutive NF-kappaB activation in AML: Causes and treatment strategies, Crit. Rev. Oncol. Hematol., 98 (2016), 35-44. https://doi.org/10.1016/j.critrevonc.2015.10.001 doi: 10.1016/j.critrevonc.2015.10.001
![]() |
[66] |
J. Zhou, Y. Q. Ching, W. J. Chng, Aberrant nuclear factor-kappa B activity in acute myeloid leukemia: from molecular pathogenesis to therapeutic target, Oncotarget, 6 (2015), 5490-5500. https://doi.org/10.18632/oncotarget.3545 doi: 10.18632/oncotarget.3545
![]() |
[67] |
C. H. Choi, H. Xu, H. Bark, T. B. Lee, J. Yun, S. I. Kang, et al., Balance of NF-kappaB and p38 MAPK is a determinant of radiosensitivity of the AML-2 and its doxorubicin-resistant cell lines, Leuk. Res., 31 (2007), 1267-1276. https://doi.org/10.1016/j.leukres.2006.11.006 doi: 10.1016/j.leukres.2006.11.006
![]() |
[68] |
A. Volk, J. Li, J. Xin, D. You, J. Zhang, X. Liu, et al., Co-inhibition of NF-kappaB and JNK is synergistic in TNF-expressing human AML, J. Exp. Med., 211 (2014), 1093-1108. https://doi.org/10.1084/jem.20130990 doi: 10.1084/jem.20130990
![]() |
[69] |
M. C. Bosman, H. Schepers, J. Jaques, A. Z. Brouwers-Vos, W. J. Quax, J. J. Schuringa, et al., The TAK1-NF-kappaB axis as therapeutic target for AML, Blood, 124 (2014), 3130-3140. https://doi.org/10.1182/blood-2014-04-569780 doi: 10.1182/blood-2014-04-569780
![]() |
[70] | M. Ma, X. Wang, N. Liu, F. Shan, Y. Feng, Low-dose naltrexone inhibits colorectal cancer progression and promotes apoptosis by increasing M1-type macrophages and activating the Bax/Bcl-2/caspase-3/PARP pathway, Int. Immunopharmacol., 83 (2020), 106388. https://doi.org/10.1016/j.intimp.2020.106388 |
[71] | N. Liu, M. Ma, N. Qu, R. Wang, H. Chen, F. Hu, et al., Low-dose naltrexone inhibits the epithelial-mesenchymal transition of cervical cancer cells in vitro and effects indirectly on tumor-associated macrophages in vivo, Int. Immunopharmacol., 86 (2020), 106718. https://doi.org/10.1016/j.intimp.2020.106718 |
[72] |
A. C. Menezes, M. Carvalheiro, J. M. P. F. de Oliveira, A. Ascenso, H. Oliveira, Cytotoxic effect of the serotonergic drug 1-(1-Naphthyl)piperazine against melanoma cells, Toxicol. Int. Vitro, 47 (2018), 72-78. https://doi.org/10.1016/j.tiv.2017.11.011 doi: 10.1016/j.tiv.2017.11.011
![]() |
[73] | G. G. Wei, L. Gao, Z. Y. Tang, P. Lin, L. B. Liang, J. J. Zeng, et al., Drug repositioning in head and neck squamous cell carcinoma: An integrated pathway analysis based on connectivity map and differential gene expression, Pathol. Res. Pract., 215 (2019), 152378. https://doi.org/10.1016/j.prp.2019.03.007 |
[74] |
J. Takezawa, Y. Ishimi, K. Yamada, Proteasome inhibitors remarkably prevent translesion replication in cancer cells but not normal cells, Cancer Sci., 99 (2008), 863-871. https://doi.org/10.1111/j.1349-7006.2008.00764.x doi: 10.1111/j.1349-7006.2008.00764.x
![]() |
[75] |
P. G. Richardson, C. Mitsiades, T. Hideshima, K. C. Anderson, Bortezomib: proteasome inhibition as an effective anticancer therapy, Annu. Rev. Med., 57 (2006), 33-47. https://doi.org/10.1146/annurev.med.57.042905.122625 doi: 10.1146/annurev.med.57.042905.122625
![]() |
[76] |
I. Zavrski, C. Naujokat, K. Niemoller, C. Jakob, U. Heider, C. Langelotz, et al., Proteasome inhibitors induce growth inhibition and apoptosis in myeloma cell lines and in human bone marrow myeloma cells irrespective of chromosome 13 deletion, J. Cancer Res. Clin. Oncol., 129 (2003), 383-391. https://doi.org/10.1007/s00432-003-0454-6 doi: 10.1007/s00432-003-0454-6
![]() |
[77] | W. X. Wang, B. H. Kong, P. Li, K. Song, X. Qu, B. X. Cui, et al., Effect of extracellular signal regulated kinase signal pathway on apoptosis induced by MG262 in ovarian cancer cells, Zhonghua Fu Chan Ke Za Zhi, 43 (2008), 690-694 |
[78] |
J. Y. Wu, S. S. Lin, F. T. Hsu, J. G. Chung, Fluoxetine inhibits DNA repair and NF-kB-modulated metastatic potential in non-small cell lung cancer, Anticancer Res., 38 (2018), 5201-5210. https://doi.org/10.21873/anticanres.12843 doi: 10.21873/anticanres.12843
![]() |
[79] | L. C. Hsu, H. F. Tu, F. T. Hsu, P. F. Yueh, I. T. Chiang, Beneficial effect of fluoxetine on anti-tumor progression on hepatocellular carcinoma and non-small cell lung cancer bearing animal model, Biomed. Pharmacother., 126 (2020), 110054. https://doi.org/10.1016/j.biopha.2020.110054 |
[80] | A. R. Mun, S. J. Lee, G. B. Kim, H. S. Kang, J. S. Kim, S. J. Kim, Fluoxetine-induced apoptosis in hepatocellular carcinoma cells, Anticancer Res., 33 (2013), 3691-3697 |
[81] | D. Sun, L. Zhu, Y. Zhao, Y. Jiang, L. Chen, Y. Yu, et al., Fluoxetine induces autophagic cell death via eEF2K-AMPK-mTOR-ULK complex axis in triple negative breast cancer, Cell Prolif., 51 (2018), e12402. https://doi.org/10.1111/cpr.12402 |
[82] |
A. M. Kabel, A. A. Elkhoely, Ameliorative potential of fluoxetine/raloxifene combination on experimentally induced breast cancer, Tissue Cell, 48 (2016), 89-95. https://doi.org/10.1016/j.tice.2016.02.002 doi: 10.1016/j.tice.2016.02.002
![]() |
[83] |
M. Bowie, P. Pilie, J. Wulfkuhle, S. Lem, A. Hoffman, S. Desai, et al., Fluoxetine induces cytotoxic endoplasmic reticulum stress and autophagy in triple negative breast cancer, World J. Clin. Oncol., 6 (2015), 299-311. https://doi.org/10.5306/wjco.v6.i6.299 doi: 10.5306/wjco.v6.i6.299
![]() |
[84] |
T. M. Khing, W. W. Po, U. D. Sohn, Fluoxetine enhances anti-tumor activity of paclitaxel in gastric adenocarcinoma cells by triggering apoptosis and necroptosis, Anticancer Res., 39 (2019), 6155-6163. https://doi.org/10.21873/anticanres.13823 doi: 10.21873/anticanres.13823
![]() |
[85] |
P. P. Khin, W. W. Po, W. Thein, U. D. Sohn, Apoptotic effect of fluoxetine through the endoplasmic reticulum stress pathway in the human gastric cancer cell line AGS, Naunyn Schmiedebergs Arch. Pharmacol., 393 (2020), 537-549. https://doi.org/10.1007/s00210-019-01739-7 doi: 10.1007/s00210-019-01739-7
![]() |
[86] | M. Marcinkute, S. Afshinjavid, A. A. Fatokun, F. A. Javid, Fluoxetine selectively induces p53-independent apoptosis in human colorectal cancer cells, Eur. J. Pharmacol., 857 (2019), 172441. https://doi.org/10.1016/j.ejphar.2019.172441 |
[87] |
V. Kannen, S. B. Garcia, W. A. Silva, M. Gasser, R. Monch, E. J. Alho, et al., Oncostatic effects of fluoxetine in experimental colon cancer models, Cell Signal, 27 (2015), 1781-1788. https://doi.org/10.1016/j.cellsig.2015.05.008 doi: 10.1016/j.cellsig.2015.05.008
![]() |
[88] | V. Kannen, H. Hintzsche, D. L. Zanette, W. A. Silva, S. B. Garcia, A. M. Waaga-Gasser, et al., Antiproliferative effects of fluoxetine on colon cancer cells and in a colonic carcinogen mouse model, PLoS One, 7 (2012), e50043. https://doi.org/10.1371/journal.pone.0050043 |
[89] |
H. Stopper, S. B. Garcia, A. M. Waaga-Gasser, V. Kannen, Antidepressant fluoxetine and its potential against colon tumors, World J. Gastrointest. Oncol., 6 (2014), 11-21. https://doi.org/10.4251/wjgo.v6.i1.11 doi: 10.4251/wjgo.v6.i1.11
![]() |
[90] | S. J. Koh, J. M. Kim, I. K. Kim, N. Kim, H. C. Jung, I. S. Song, et al., Fluoxetine inhibits NF-kappaB signaling in intestinal epithelial cells and ameliorates experimental colitis and colitis-associated colon cancer in mice, Am. J. Physiol. Gastrointest. Liver Phys., 301 (2011), G9-19. https://doi.org/10.1152/ajpgi.00267.2010 |
[91] |
K. H. Liu, S. T. Yang, Y. K. Lin, J. W. Lin, Y. H. Lee, J. Y. Wang, et al., Fluoxetine, an antidepressant, suppresses glioblastoma by evoking AMPAR-mediated calcium-dependent apoptosis, Oncotarget, 6 (2015), 5088-5101. https://doi.org/10.18632/oncotarget.3243 doi: 10.18632/oncotarget.3243
![]() |
[92] |
J. Ma, Y. R. Yang, W. Chen, M. H. Chen, H. Wang, X. D. Wang, et al., Fluoxetine synergizes with temozolomide to induce the CHOP-dependent endoplasmic reticulum stress-related apoptosis pathway in glioma cells, Oncol. Rep., 36 (2016), 676-684. https://doi.org/10.3892/or.2016.4860 doi: 10.3892/or.2016.4860
![]() |
![]() |
![]() |