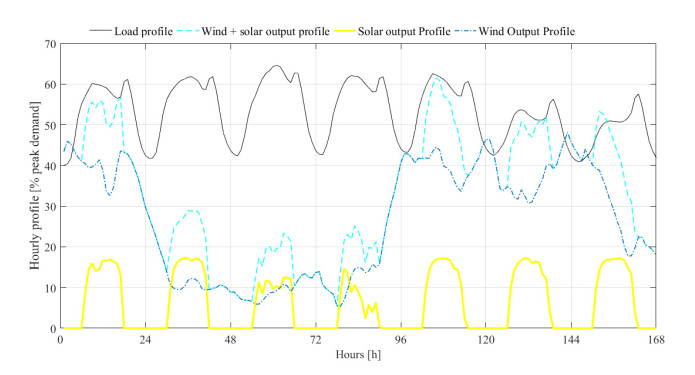
Citation: Solomon A. A., Michel Child, Upeksha Caldera, Christian Breyer. Exploiting wind-solar resource complementarity to reduce energy storage need[J]. AIMS Energy, 2020, 8(5): 749-770. doi: 10.3934/energy.2020.5.749
[1] | Mohamed Hamdi, Hafez A. El Salmawy, Reda Ragab . Optimum configuration of a dispatchable hybrid renewable energy plant using artificial neural networks: Case study of Ras Ghareb, Egypt. AIMS Energy, 2023, 11(1): 171-196. doi: 10.3934/energy.2023010 |
[2] | Eugene Freeman, Davide Occello, Frank Barnes . Energy storage for electrical systems in the USA. AIMS Energy, 2016, 4(6): 856-875. doi: 10.3934/energy.2016.6.856 |
[3] | Rashid Al Badwawi, Mohammad Abusara, Tapas Mallick . Speed control of synchronous machine by changing duty cycle of DC/DC buck converter. AIMS Energy, 2015, 3(4): 728-739. doi: 10.3934/energy.2015.4.728 |
[4] | Nadwan Majeed Ali, Handri Ammari . Design of a hybrid wind-solar street lighting system to power LED lights on highway poles. AIMS Energy, 2022, 10(2): 177-190. doi: 10.3934/energy.2022010 |
[5] | Jorge Morel, Yuta Morizane, Shin'ya Obara . Seasonal shifting of surplus renewable energy in a power system located in a cold region. AIMS Energy, 2014, 2(4): 373-384. doi: 10.3934/energy.2014.4.373 |
[6] | Virendra Sharma, Lata Gidwani . Optimistic use of battery energy storage system to mitigate grid disturbances in the hybrid power system. AIMS Energy, 2019, 7(6): 688-709. doi: 10.3934/energy.2019.6.688 |
[7] | Malhar Padhee, Rajesh Karki . Bulk system reliability impacts of forced wind energy curtailment. AIMS Energy, 2018, 6(3): 505-520. doi: 10.3934/energy.2018.3.505 |
[8] | Arben Gjukaj, Rexhep Shaqiri, Qamil Kabashi, Vezir Rexhepi . Renewable energy integration and distributed generation in Kosovo: Challenges and solutions for enhanced energy quality. AIMS Energy, 2024, 12(3): 686-705. doi: 10.3934/energy.2024032 |
[9] | D.M. Reddy Prasad, R. Senthilkumar, Govindarajan Lakshmanarao, Saravanakumar Krishnan, B.S. Naveen Prasad . A critical review on thermal energy storage materials and systems for solar applications. AIMS Energy, 2019, 7(4): 507-526. doi: 10.3934/energy.2019.4.507 |
[10] | Khuthadzo Kgopana, Olawale Popoola . Improved utilization of hybrid energy for low-income houses based on energy consumption pattern. AIMS Energy, 2023, 11(1): 79-109. doi: 10.3934/energy.2023005 |
An increased use of variable renewable energy (VRE) resources, such as wind and solar energy, has created a concern over the corresponding challenges of their intermittency. One of the major challenges relates to reserve requirements to address intermittency and the ability to enhance grid matching capability. A recent article [1] provides a review of the different challenges and possible solutions to tackle the significant technical level challenges that accompany high penetration of photovoltaic (PV) generation in the energy system. Technical solutions, such as demand response, the use of energy storage technologies, and excess energy curtailment were expected to ameliorate these challenges. Reports show that demand response could improve load following capability of the power systems [2,3,4]. Energy storage also has the potential to improve grid flexibility and increase grid penetration of variable renewable energy resources while curtailment was reported to lead to high penetration at reduced storage and conventional balancing resources [1,5,6,7,8,9,10,11,12,13,14,15,16,17,18,19,20,21,22,23]. In a recent study, the link between curtailment, penetration and storage need was reported to play a significant role in system design during energy transition [24]. Other studies examined the possibility of overcoming the challenge that comes with wind-solar variability by exploiting its complementarity to increase the energy penetration of these resources and mitigate the associated operational challenges [6,20,25,26,27,28,29,30,31,32,33,34,35,36,37,38,39,40,41,42,43,44,45,46,47]. Particularly, the study by [6] reported that resource complementarity carries multi-dimensional benefits to the system. Even though there is a clear consensus that complementarity of resources, such as wind and solar technologies, leads to a smoothing effect that may reduce ramping requirements and transmission congestion while also significantly improving grid matching of these resources [4,5,20,22,23,24,25,26,27,28,29], the magnitude of this benefit against other measures and how this could be implemented during system design is not yet clear.
The benefits of complementarity were reported in 6 groups of studies. The first group [25,26] approaches the question from meteorological perspectives, applying techniques such as correlation tests, standard deviations and other indicators between diverse resources as well as geographic locations. The second group [27,28,29] applies almost similar techniques to evaluate complementarity between power generated by different resources and at different locations. Typically, both types of studies report negative correlation between wind and solar resources (which was interpreted as the presence of resource complementarity), while also reporting location dependent spatial complementarity. Other than showing the potential benefits, these studies are insufficient to specifically quantify the benefits economically or technically. A few studies that quantified economic or technical benefits of complementarity exist [5,6,7,9,20,30,31,32,33,34,35,36,37,38,39,40]. The remaining four groups fall in these categories. The third group are those trying to quantify the benefit, in terms of economics and use optimisation models [9,30], while comparing some specific cases of resource complementarity with any of the stand-alone systems of the target resources. While such studies report economic benefits of complementarity, their ability to clearly show the technical benefit is limited. The fourth group of research evaluated the benefit of wind-solar complementarity to improve system reliability using a loss of load parameter [43] and other indicators such as firm capacity [32]. The fifth group of studies [5,6,32,33,34] has evaluated the technical benefits of complementarity using various indicators, typically grid matching (penetration), impact on storage and balancing capacity requirement, etcetera. The sixth group deals with the subject based on data gathered from utilities to quantify the role of complementarity as currently experienced by utilities [35]. Such studies provide important lessons as regards to its role in the operation of existing systems, particularly in decreasing reserve requirement. However, due to the present low penetration of VRE in most countries, the ability to obtain full operational lessons is still limited. More importantly, lessons that will inform design level decisions may come from more diversified studies that are built on understanding system characteristics than an analysis of the existing system. Thus, it is important to develop a broader view. In this study, we will examine the various advantages that complementarity could provide to a future grid in general and its ability to reduce storage requirements.
The above studies vary in scope, geographic location, and methods even in the same groups given above. They also vary in the number of resources they evaluate. Some try to find benefits of spatial distribution using one resource or more, others examine complementarity between various resources regardless of geographic distribution [22,25,26,27,28,29,30,31,32,33,34,35,36,37,38,39,40,41,42,43,44,45]. For instance, while wind-solar complementarity studies are common, studies looking into the complementarity of these resource to other renewables such as hydropower [33,38,40,41,42,44], bioenergy [48] and marine energy [31] also exist. Despite the varying methods and scopes, by context all these studies refer to the complementarity of generation resources to supply electricity demand. Thus, in this paper resource complementarity is defined as the ability of a spatio-temporally distributed mixture of renewable generation resources to enable better electricity supply conditions at reduced operational challenges and need of enabling technologies due to the improved matching of outputs to demand profiles. Relevant review papers dealing with the subject heavily focus on the methods and indicators used to evaluate complementarity as in [49], the definition of the concept and indicators as in [50], analysing the smoothing effect of complementarity [51], etcetera. This paper focuses on analysing and clarifying the multi-dimensional benefits of wind-solar complementarity in the power system. The paper also evaluates how these benefits are manifested in various studies and how these potentials could be exploited during system design. To get better insights, results of studies performed at hourly resolution were closely examined. Power system level studies that allow an easy comparison of various conditions were given due attention for this analysis.
Considering wind and solar resources, electrical output profiles vary significantly depending on geographic location, weather, time of the day, season and various topographic conditions. Figure 1 presents a one-week agammaegated simulated hourly output profile of wind and solar plants, and the corresponding demand profile for California. The total capacity of the wind-solar hybrid system in this simulation was 40.9 GW (11.1 GW solar and 29.8 GW wind). The system was distributed throughout California and would have supplied the maximum possible energy (38.3% of the annual demand) that California could have obtained from intermittent renewables in the year 2011 without energy dumping or storage. Note that achieving the same penetration with solar or wind technologies alone would have required some curtailment, storage or both.
As shown in Figure 1, solar generation gradually increases until noon, when it starts the opposite gradual decrease during the afternoon on a typical sunny day (see days 5–7) [6]. Cloud cover adds more solar output variability on cloudy/semi-cloudy days, as shown for days 1–4, by temporarily reducing/blocking the received solar irradiance depending on the relative size of the PV system and the cloud. Unlike solar, wind has a less defined diurnal output profile but for most geographic places, it is reported to reach its peak generation during the evening time. However, if one regards the total output over the day, wind tends to demonstrate some sort of periodic output, i.e. a few continuous days of good generation (which depends on the season) before/after days of poor generation, unlike solar (which does not show the spikey nature of wind output to be discussed below).
The diurnal behavior of both resources seems common to most places, depending on local weather. However, seasonal variability and resource quality show significant differences from place to place. This can be seen by comparing Figures 2, 3 and 4, which present seasonal variability of wind and solar energy production for California [6], Finland [10] and the Kingdom of Saudi Arabia (KSA) [11], respectively. The daily output shows that wind output peaks in spring for California and KSA, and in wintertime in Finland. Due to its tendency to show some periodic trend in terms of its daily generation, wind output is very spiky, showing several dips after reaching peak daily output. Overall wind output in Finland is poor in the summer as compared to other seasons. The difference in seasonal variation of the solar output between Finland and the two places is even larger.
As shown in Figure 3, Finland’s solar output is almost zero from November through February, the diurnal variation in summer also indicates that it is affected by cloud cover. On the contrary, the solar generation in California and KSA appears smoother during summer and provides good generation during winter depending on local weather (such as cloud cover). Note that the output presented corresponds to a VRE penetration of 70% of total annual energy demand of Finland, and 38.1% and 98% of the annual electricity demand of California and KSA, respectively. Thus, the comparison should focus only on the diurnal and seasonal profile rather than its relative value as compared to the corresponding daily average demand. The often higher than demand generation in Finland and KSA occurred because of the energy storing process using technologies such as batteries, synthetic natural gas storage and sector coupling (heat, gas, transport). In contrast, in Figure 2 where only the power sector of the state of California had been examined at lower penetration, daily generation did not exceed demand because the size of the variable renewable system is lower.
The above change in resource quality and profile is common even at smaller differences in geographic location. Particularly, the presence of mountain terrain may result in a significant observed output difference of these resources at a given time of the year. However, the difference between locations with small geographic separation over some season may be small, particularly for solar.
The foregoing discussion shows significant diversity in the output profiles of wind and solar technologies. This may bring significant benefit to the future energy system through smoothing and improved grid matching that in turn result in high grid penetration, reduced energy storage and balancing capacity needs, improved reliability, etcetera. Though the hybrid is always better, its smoothing impact will not remove extreme ramping requirements at some time of the year that may still be a challenge (see for example the down ramp of the hybrid system starting at the end of days 1 and 7; and the up ramp starting on the evening of day 4 of Figure 1). However, the benefit in increasing penetration and reducing storage could be significant. Returning to Figure 1, one can see that the solar/wind hybrid system matches the load profile better than the respective stand-alone systems. If the system size is doubled, assuming approximately similar profiles, generation will be higher than the load (which requires storage or another spilling means). This becomes more of an issue of concern than balancing the demand during the week.
In the following subsections, we will examine the potential benefits of complementarity and how exploiting it could benefit the future energy system. Due to the challenge of creating simplified comparative criteria between various studies, we will focus on making comparisons based on grid penetration, storage size, and share of wind or solar as percent of total VRE capacity, balancing capacity, etcetera.
Several studies evaluating the benefits of complementarity from a meteorological perspective or from power generated by solar/wind resources have concluded that variability is smoothed, which may lead to a decrease in ramping requirement. The smoothing effect is usually measured using correlation tests, with negative correlation being interpreted as the presence of complementarity. Complementarity in this case could be understood as the measure of high degree of VRE output distribution over time, which reduces the number of times over which energy is not generated by the combined wind and solar resource. Solar was found to show strongest correlation in most reports, which weakly decreases as distance between resource location increases [32]. Similarly, wind resources show relatively strong correlation that decreases a bit faster with increased separation distance, indicating some degree of complementarity [32]. Such complementarity is usually credited as a factor that smooths the overall wind energy output to be injected into the power system [25,26,27,32,55]. The consequence of that effect is reduced ramping requirement and reserve capacity need. More importantly, mixing solar and wind resource as well as increased spatial distribution was linked to weak anti-correlation [25,27,32] and reduced variability [52,53,54], which confirms the presence of complementarity between the two resources. The effect of such complementarity not only reduces ramping and reserve needs but also enables the possibility of increased grid penetration of wind-solar hybrid systems as compared to one of them as a stand-alone [6,22,34]. It also improves the reliability of VRE as energy supply resources, even though it does not fully solve its fundamental challenges [32,35,43,55]. The issue of reliability is discussed in the next section in order to focus on its effect on the two challenges, namely, ramping need and grid penetration.
Ramping is a rapid change in power output of the dispatchable generators induced by the variability of both generator (wind and solar) output as well as the electricity demand. An increase in ramp is usually termed a ‘ramp up’ while its decrease is known as a ‘ramp down’. Due to the strict need to maintain the generation and demand balance within technically prescribed limits to enforce power system security criteria, operators need to ensure the presence of sufficient load following resources to compensate for such variations. With the integrations of variable renewable resources, particularly wind and solar, the flexible load following resources must balance any change in net load. Net load is load minus the VRE output. Thus, the ramping caused by the added VRE resources is usually quantified by studying the net load ramp.
All studies that analysed the impact of integrating VRE resources on power system ramping need showed that ramping need and frequency increases as a result of the added variability caused by heavily weather dependent energy generation technologies [21,29,35]. The ramping need was reported to depend on VRE penetration, its mix and the geographic scale of the system. For low penetration, the ramping need could be compensated by the resources that are prepared to manage the regular fluctuation of the power system. However, as the penetration increases, the ramping need was reported to significantly increase, requiring more flexible load following resources: either generators, storage units, or more comprehensive power line interconnections. Net load ramping magnitude related to solar PV increases very strongly as PV system size increases, but the frequency remains lower due to more than 50% zero hours at night [21,29]. On the other hand, the wind related net load ramp increases both in magnitude and frequency [29,35,55]. The wind-solar mix also shows similar behaviour, but the extreme ramp event increases with PV share as observed for Europe [29]. The geographic distribution was reported to ameliorate the ramping need, particularly for wind resources [29,35,55]. A study made using actual measured data shows good agreement with the above findings while also adding interesting insights [35,55]. The study, which analysed the impact of actual variable renewable generation on actual net load ramp in California, shows that the frequency and uncertainty in system level net load ramp increased during 2016. At the same time, the average ramp up and ramp down magnitude was found to decrease as compared to the regular load ramp. The decrease was due to the observed implicit ramping compensation that renewables provided at some of the challenging regular ramping hours. Specifically, the net load ramp up and down magnitude was reduced during morning and evening hours, respectively, due to the corresponding down ramping trend of solar and an up ramping trend of wind during the evening that may have compensated for some of the evening up ramp. Note that the study qualifies a ramp event as 5% of the total load, which may exclude extreme events.
In another study that analysed several countries’ actual wind generation data, it was shown that regions with better wind distribution report lower ramping as a percentage of wind capacity [55]. Data based on simulated results also show that wind-solar complementarity has the potential to ameliorate related ramping need [29]. In addition to exploiting such resource potentials, increased cooperation between adjacent regional power systems can also be used to reduce the balancing need by sharing resources [55,56]. At the same time, wind and solar resources may also participate in the process of providing balancing, particularly at a time that requires curtailment [57]. However, even if the ramping challenge is managed well at all penetrations, the amount of penetration to be achieved without storage and curtailment will be limited regardless of wind-solar complementarity. Very high energy penetration without storage requires a significant amount of curtailment and vice versa.
With regards to the impact of wind-solar complementarity on grid penetration, reports show that VRE hybrids resulted in a significant increase in grid penetration as compared to any one of them as a stand-alone. Table 1 shows an estimate of grid penetration for solar capacity shares of 0%, 50% and 100% of the total VRE system capacity for two scenarios of curtailment. The estimates show significant improvement in grid penetration for the wind-solar mix as compared to the wind or solar technologies as a stand-alone. This is due to the direct effect of the wind-solar resource anti-correlation that dramatically improved grid matching. Better penetration for the case of California’s grid was partly due to the role of transmission lines plus the geographic distribution of resources.
Country/region | Share of solar technology in the total VRE capacity | |||
0 | 0.5 | 1 | ||
Penetration (% of annual demand) | Israel (5% curtailment) | 32 | 40* | 29 |
Israel (0% curtailment) | 17 | 19 | 17 | |
California (~0% curtailment) | 26 | 36+ | 27 | |
California (~5% curtailment) | 40 | 52 | 41 | |
+: The optimal mix gives approximately 38.2%; *: Penetration reaches 46% for concentrating photovoltaic (CPV) technologies. |
In Shaner et al. [34], a similar grid matching effect was investigated over a larger geographic region covering the continental United States and by fixing the share of energy generated by solar or wind as a percentage of the total VRE generation (as opposed to evaluating at fixed capacity share as summarised above). At the same time, that paper refers to ‘the calculated energy penetration (the percent of the electrical energy demand met by VRE)’ as reliability even though several articles use the term (energy) penetration [2,20]. In the specific area of power systems, the term ‘reliability’ represents a broader context that deals with system adequacy and system security. System adequacy, which may have the same relation with energy penetration, is usually measured by indices such as loss of load expectation, loss of load probability, expected energy not served, frequency and duration of forced outage [58]. Most of these indicators are probabilistic and they measure that the expected shortcomings are within an allowed range as opposed to energy penetration. Thus, energy penetration is used to summarise their results in a way that is consistent with other sources and the meaning of the term.
Table 2 summarises the approximate values of three parameters estimated after analysing the data presented in [34]. Specifically, the estimate is based on their Figure 3. To simplify the estimation, a point on a curve is chosen so that the mean VRE generation ratio to the demand (y-axis) is approximately 1 and meets the corresponding penetration (‘reliability’) curve for the case of their no-storage scenario. This allowed us to easily estimate the corresponding curtailment value. The data presented for all cases show high penetration due to the corresponding large curtailment as compared to the above two studies. The data in [6] shows approximately 75% penetration of the wind-solar mix at 25% curtailment of the Californian grid, which is in good agreement with this data. At the same time, the large geographic swath may contribute some value though that appears very small in the present condition. Overall, it can be concluded that wind-solar complementarity not only increase VRE penetration but also reduces the amount of curtailment that is needed to achieve that penetration as compared to the wind or solar system as a stand-alone. However, it is very important to note that high penetration without storage requires a wind dominant system because of the hourly distribution of its output as compared to solar.
Solar share (% total generation) | Penetration (% annual demand) | Curtailment (% generation) | Remark |
0 | ~75 | ~25 | Though the 25% wind share achieves approximately the same penetration at equal curtailment as compared to the 100% wind system, note that it required lesser areas of aggregation. For the same aggregation area, Figure 2 suggests that penetration and curtailment corresponding to a 100% wind energy generation mix is approximately 70% and 30%, respectively. |
25 | 75 | 25 | |
50 | 73 | 27 | |
75 | 60 | 40 | |
100 | 50 | 50 |
Note that the high curtailment in Table 2 is due to the selected reference point in Figure 3 of [34] but not to endorse high curtailment. A result from well optimised techno-economic studies does not show a curtailment higher than 15% [24]. The curtailment in Table 2 is even higher when it is compared to total loss (curtailment plus storage loss) in several other studies that are reporting a VRE penetration value higher than 85% [6,17,18,19]. More importantly, techno-economic studies that consider diverse resources have shown lower curtailment values of 2.4% to 7.2%, depending on scenario design and system structure [59,60,61,62]. However, because curtailment and storage loss show substitutional effects [24] depending on system design and assumed operation, it is better to use total loss as a comparative indicator, which is found for [60] at a global average of 11.2% for a global cost-optimised 100% RE power system based on 145 regions with no cross-border interconnection power lines.
The foregoing discussion shows that wind-solar complementarity smooths the output and increases grid penetration of the intermittent resources while reducing ramping requirements. However, its ability to achieve very high penetration will still be dependent on the presence of enabling technologies such as energy storage. The upcoming section summarises storage need and how it depends on complementarity.
Impacts of complementarity on energy storage were examined in studies by [5,7] for the European power system and [6] for the Californian power system. Due to significant differences in underlying assumptions and methods as well as reporting, a detailed comparison was difficult. However, both studies agree that optimal complementarity reduces storage need and balancing (backup) capacity requirement. Moreover, they have shown that, depending on the amount of allowed energy curtailment, further significant reduction in energy storage and balancing capacity requirement was possible. Focusing on the impacts of complementarity, the European study has shown that relative to wind/solar as a stand-alone, an optimal mix of the two technologies reduce the storage need for 100% VRE penetration by a factor of 2. The study by [6] looks into the question in a far more detailed way and as a result shows several interesting features that need to be discussed. Below, we present a brief summary of that result.
Figure 5 shows that solar and wind have significant differences in the storage capacity requirement and use. Note that these studies [5,6,7,17,18,19] are focused on solar PV and solar CPV, whereas concentrated solar thermal power (CSP) plants without storage were included only in [6]. As energy storage capacity (with negligible energy loss) increases, penetration initially increases almost linearly for all wind-solar mixes before they level off (see Figure 5). Solar results in less storage, and due to its almost diurnal charging and discharging cycle, it uses the storage more efficiently in a technical sense. As discussed above, because of the longer periodic cycle of good and weak wind generation days, there is a requirement for larger storage (hence larger energy to power capacity ratio) to reach almost the same level of penetration as solar. This results in lower storage utilisation. In a recent study of storage requirement for increased VRE penetration [24] performed using a techno-economic model, the nexus between curtailment-storage-penetration was explained. The study shows that the three parameters simultaneously increase during energy transition. The fundamental findings of this study, which were explained using the similar trends reported in [17,19], show that a storage capacity lower than daily average demand suffices to reach a grid penetration of approximately 90% of the annual demand. These storage technologies are classified as diurnal storage. However, to arrive at VRE penetration above 90% a different class of storage, which is classed as seasonal storage, should be added in significant magnitude, so that cost-optimised solutions can be obtained [24]. Data presenting similar results [11,12,23,42,59,60,61,62,63,64] are good evidence that the nexus exists regardless of resource mix but evidently the specific optimal curve depends on the potential resource mix that can be achieved depending on specific conditions. For high penetration, high wind or its stand-alone system may require more curtailment or storage capacity to arrive at the same penetration depending on which is more economical as compared to solar dominated systems.
The disadvantage of a wind dominated or a stand-alone wind system was also observed on the balancing conventional technology requirement, which is also termed as backup in non-100% RE systems. In short, wind requires the largest balancing capacity as compared to the wind-solar hybrid or even solar as a stand-alone. For an easy comparison, Tables 3 and 4 are presented in the following. Table 3 presents VRE penetration and the required balancing capacity by keeping storage the same for two energy loss scenarios. Table 3 shows that resource diversity converts the combined effect of storage and energy curtailment to an opportunity in order to arrive at higher penetration with smaller storage. Note that wind persistently shows disadvantages in the achieved grid penetration and reducing balancing capacity requirements, while solar has shown a good advantage in reducing balancing capacity in both scenarios. Due to this, it was reported that in resource rich regions, depending on the relative cost of technologies, target penetration and the respective resource qualities, the mix could be dominated by any one of the resources. Several studies have reported similar results, though using varying cost estimates, levels of curtailment, wind-solar mix and storage size [4,5,6,10,33,34]. This shows that the optimal wind-solar mix should be determined according to the best match with the local load and other technical benefits to the respective energy system while considering economic requirements. From the preceding section, it is clear that at lower penetration, where storage application is less important, wind dominated systems show an advantage by achieving relatively higher penetrations.
Total energy loss (% of VRE generation) | Parameters | Solar share of total VRE by capacity | Storage energy capacity | ||
0% | 50% | 100% | |||
0% | VRE penetration (% of annual demand) | 38 | 58 | 52 | 300 GWh |
Required balancing capacity (GW) | 56 | 47 | 46 | 300 GWh | |
15% | VRE penetration (% of annual demand) | 55 | 81 | 62.9 | 186 GWh |
Required balancing capacity (GW) | 52 | 31 | 39 | 186 GWh |
Total VRE capacity (GW) | Penetration (% of annual demand) | Network energy capacity (GWh) | Network power capacity (GW) | Balancing capacity (GW) | Wind (% of total VRE capacity) |
65.5 | 52.6 | 4730.6 | 44.4 | 50.3 | 100 |
58.2 | 51.9 | 424.4 | 35.8 | 50.3 | 75 |
58.2 | 52.1 | 320.2 | 22.2 | 50.7 | 70 |
58.2 | 52.2 | 232.2 | 20.6 | 51.3 | 65 |
58.2 | 52.3 | 201.3 | 21.7 | 51.4 | 60 |
58.2 | 52.3 | 165.9 | 19.4 | 51.5 | 55 |
58.2 | 52.2 | 154.2 | 18.1 | 51.7 | 50 |
58.2 | 52.1 | 153.8 | 18.4 | 51.3 | 45 |
58.2 | 51.8 | 160.6 | 18.7 | 51.2 | 40 |
67.6 | 51.9 | 322.3 | 29.0 | 48.3 | 0 |
Table 4 presents technological capacity requirements to arrive at a grid penetration of approximately 52.5 ± 0.5% of the annual demand for the case of no energy loss. From this table, one can see that complementarity brings significant advantage over any of the stand-alone systems. It also shows that the storage requirement for wind and solar as stand-alone systems were approximately 30 and 2 folds the lowest storage capacity (which is 154 GWh), respectively. Other researchers [5,7] have reported that storage capacity requirement for wind as a stand-alone reduced by half due to complementarity, as reported for a simplified 100% power system analysis solely based on wind and solar. This suggests that the comparative advantage of the wind-solar mix in reducing storage may depend on grid penetration, among other things. As can be seen in Figure 5, after the initial small linear increase of grid penetration with storage, where each wind-solar mix shows almost comparable penetration for energy storage, each mix starts to show varying degrees of penetration for a single type of storage, with wind showing the lowest and solar the highest penetration. The above US-wide theoretical complementarity study [34] also evaluates the situation under the availability of the same storage capacity. The result shows that when solar contributed 70–75% of the wind-solar generation, a higher wind-solar penetration was achieved with 12 hours of storage. Similarly, in [6] higher penetration was achieved by a solar dominated system and a lower storage capacity for California. This effect is a consequence of a fundamental matching effect of these resources with [20,22] and without [17,19] storage to the local demand profile. Tables 3 and 4 also show that for up to 90% grid penetration (depending on allowed total energy loss), solar arrives at a higher grid penetration with smaller storage capacity as compared to wind. However, beyond 90% it starts to require a significantly larger storage size due to the need for seasonal storage capacity [24]. At 100% grid penetration, solar needs a very large storage capacity, which is comparable to what is required by wind. In general, one can conclude that wind-solar resource complementarity brings the significant advantage of reducing energy storage capacity and balancing capacity requirements to arrive at higher penetration. As shown in this comparison, the comparative reduction in storage size requirement depends, inter alia, on grid penetration.
Economic models were also used to show the advantage of complementarity, but technical lessons such as the one discussed above are important to better understand key physical limitations in economic models based on constraints. Even if a defined trend in storage requirement as a function of resource mix is lacking, the benefit of resource diversity is clear from several reports. Specifically, considering broader resource complementarity, it is possible to see further reduction of storage capacity requirements by adding other renewable technologies. Resource complementarity between wind-solar and hydropower [30], and wind-solar and tidal barrage [31] were also reported. Other renewables, such as those based on bioenergy, could also complement these resources [48]. In transitioning to 100% renewable energy, exploiting resource complementarity carries an advantage of arriving at a more efficient and perhaps sustainable energy system. In the upcoming subsection, the role of broader resource complementarity will be briefly discussed.
As discussed above, reliability is measured at a power system level [58]. The relevant metrics are Loss of Load Probability (LOLP) or Loss of Load Expectation (LOLE), which represent the probability of an outage and the expected hours of outage per year, respectively [65]. The parameters typically take maximum restriction limits of about 0.05% and 3 h/year for LOLP and LOLE, respectively [65]. Based on current knowledge, it is impossible to comply with such requirements by relying on wind or solar as a stand-alone or their hybrid because of the low matching capability, without further technical solutions. Complying with such limits will require, among other things, enabling technologies, such as storage, power line interconnections, sector coupling, and curtailment. Data in this study show that the wind-solar hybrid system supplies a little over 70% of the annual demand with large curtailment. However, this is not to say that wind-solar complementarity does not improve reliability. This evidence can be understood from the possibility to reduce times of power unavailability and improve firm capacity and peak average capacity percentages through resource complementarity. The reduction in times of power unavailability through wind-solar complementarity can be seen from the studies covering the cases of Israel [20], the entire US [34], California [6] and ERCOT Texas [32]. Its impact in firm capacity as well as peak average capacity percentage can be seen in [32] for the case of the ERCOT Texas region. A firm capacity of as high as 17% was reported for the wind-solar mix as compared to 4% for wind and 0% for solar as a stand-alone. These facts indicate that wind resource complementarity improves the resource contribution to the capacity. But the most important question of reliability in the future system may not be about capacity but about managing the energy supply need during times of weak solar and wind generation [6]. Thus, complementarity with wider renewable resource types may be more beneficial to overcome such a problem. In the next section, it will be examined how complementarity with other resources benefits the system.
Table 5 provides typical data based on results of various studies covering different places. Note that the energy storage capacity was given in terms of the local daily average demand for easy comparison. The table shows that 100% grid penetration of VRE either takes massive energy curtailment plus smaller storage or massive energy storage and relatively lower curtailment, which may go along with declining marginal energy storage cost, as for the case of Saudi Arabia. In a study by [24], it was shown that during the energy transition an optimal link between storage energy capacity, curtailment and VRE penetration exists. However, in a very diverse system both storage and curtailment could be minimised or even avoided depending on the mix with other resources, in particular hydropower but also bioenergy and their flexibility [13,33,41,42,45,48]. In addition, in order to utilise storage and generation resources efficiently, it is always preferable to pursue a strategy that promotes an energy system that relies on diverse RE resources than to go for 100% VRE. A 100% VRE system is rather of theoretical nature for practically all countries globally, since most countries have access to some hydropower, but also to sustainable bioenergy and waste-to-energy resources, which are often dispatchable. Besides utilising other sustainable renewable resources, increasing power exchange by transmission interconnections with other regions may be necessary to overcome resource scarcity, or to improve economics.
Parameters of interest | Region/country of the study | ||||||
Finland integrated [10] | KSAa integrated [11] | KSAa [11] | Israel [17] | California [19] | Europe [5] | PJMb [9,47] | |
VRE penetration (% of annual demand) | 70 | 99 | 98 | 90 | 85 | 100 | 100 |
Energy storage capacity (GWh) | 3990 | 43407 | 45398 | 113 | 186 | 16,000 | 891 |
Energy storage capacity (daily average demand) | 8.6 | 16.5 | 19.4 | 0.83 | 0.22 | 1.8 | 1.2 |
Total energy loss (% of total VRE generation) | 6 (2.5% storage loss) | 13.4 (9% storage loss) | 14.6 (10% storage loss) | 20 (12% storage loss) | 20 (3% storage loss) | 50c | > 50c, d |
Annual demand during the studied year (TWh) | 105 | 729 | 650 | 50.2 | 302 | 3240 | 276 |
Usefulness index (a.u.) | 6.3e | 11.6e | 10e | 186 | 220 | NAf | 24 |
Storage efficiency | mixg | mixg | mixg | 75% | 75% | 100% | 81% |
Share of other RE resources (% of annual demand) | 30 (hydro, biomass) | 1 (geothermal) | 2 (geothermal) | 10h | 15h | 0 | 0 |
Wind share (% of total VRE by capacity) | 54 | 39 | 40 | 0 | 43 | 60 | 93 |
Energy sectors investigated | power, thermal sector | power, desalination | power | power | power | power | power |
a: Data correspond to the result of year 2050; b: This is based on the GIV storage scenario, which was selected for data completeness; c: Estimate may not include loss due to storage efficiency; d: Estimate based on the given data [9], in [47] it was shown that at 95% penetration the loss was 51%; e: UI could be lower for low efficiency storage because of the resulting larger energy storage need, UI for KSA was readjusted for gas storage (the improvement in UI was to maximum 5 points). For Finland, direct use of synthetic gas makes it less important (but even if necessary, the change will be a maximum 5 points); f: At 50% curtailment, for a diverse resource such as theirs, a penetration of approximately 80% is possible, one could then see the UI will at maximum be 40.5; g: Resource type not specified, but considered dispatchable renewable technologies (at the same time, data also show 98% VRE penetration at 25% total energy loss as well as storage capacity of about 1.3 and 6 times the daily average demand of California and Israel, respectively; h: The dominant capacities and their efficiencies are power-to-gas (electrolyser, 61% [10], 77% [11], CCGT 58% and OCGT 43%), battery (>90%), thermal energy storage = 90%. |
Table 5 shows how broader complementarity reduces storage requirement even when it is based on models that are not optimised. Excluding those that promoted highly renewable energy systems with extensive curtailment, the table shows that studies that considered the mix from other (non-VRE) resources have achieved very high penetration at significantly smaller storage requirement. A very good example is the case of the study of the Brazilian system [33], which reported 100% RE without the need of new storage and curtailment but relied on existing reservoirs and resource diversification by 2050. In that study, the share of solar PV, wind energy, hydropower and biomass power were 27%, 16%, 34% and 23%, respectively. The other examples are the Finnish system [13] summarised in Table 5 and the West African power system [41,42]. The storage in the case of the Finnish system is dominantly gas storage, which may be due to biogas digesters and synthetic natural gas, resulting in a large capacity at low penetration since the gas storage is mainly used for seasonal storage. The two scenarios for the KSA study [11] (Table 5) present little benefit in terms of decreasing storage capacity from sector coupling for the case of the power plus desalination scenario because the coupled water desalination sector relies on plants that have high capital cost and limited dispatch flexibility, which are not due to technical but economic restrictions [66]. The observed energy storage capacity difference between the two scenarios was dominantly due to gas storage. At the same time, the corresponding large storage capacity was also due to the requirement for large seasonal storage, which is provided by gas storage for mainly synthetic natural gas, at the achieved VRE penetration. The foregoing discussion indicates that if an optimal power system is designed to accommodate VRE, storage may have lower energy capacity and an improved service to the system as discussed in [16,17,18,19,24,31]. Under assumed broader resource complementarity, the largest storage required to reach a 100% renewable power system will approximately be at maximum daily average demand if coupled with modest energy curtailment. In cases where resource complementarity prevents this, the need for seasonal storage increases as VRE penetration starts to exceed approximately 90% of the annual demand [24]. In this condition, storage could reach as high as 30 times daily average demand depending on the resource and the final amount of VRE penetration. Resource complementarity and diversity also increase energy security, as the availability dimension is positively affected, also documented in a recent energy security index paper [67]. At the same time, in the future system the corresponding technical challenges of variability can be ameliorated using different techniques depending on VRE penetration. For example, ramping can also be managed by a mix of technologies such as hydropower, gas turbines, and batteries, as well as electrolysers [68], smart charging and vehicle-to-grid [69,70] solutions, depending on the system. Finally, even though the complementarity subject is focused on the generation technology, it is very important to note that sector coupling could bring a benefit of its own to the future system which could help in reducing some of the challenges of VRE integration [62], while it may have an influence on the relative complementarity of wind and solar.
Policymakers and system planners have to consider economic solutions, while not compromising system reliability and security. This leads to cost-optimised solutions which benefit from wind-solar complementarity, as detailed in previous sections. However, the meteorological complementarity of wind and solar, and their match with the load are not the sole factors determining cost optimization.
Cross-border grid interconnections can have an influence on the cost-optimised complementarity of wind and solar as Child et al. [56] found for the case of Europe. For the case of Europe separated in 20 regions, the cost-optimised solution led to a generation option of 39.5% wind generation and 60.5% solar PV generation, while a fully interconnected Europe led to an increase of wind generation capacities of 9.4% and a reduction of solar PV capacities of 13.5%, while total system cost was reduced by 8.6%, curtailment by 32% and total energy storage capacity by 22%. Pan-European grids finally allow a better balancing of wind energy over a larger region, so that the meteorological complementarity is shifted towards higher shares of wind energy.
Relative changes of the power generation cost of wind electricity and solar PV electricity, and supporting storage technologies such as batteries, can also have a strong impact on the cost-optimised balance of wind-solar complementarity as documented by Bogdanov et al. [60]. They describe a global power sector transition from the status as of 2015 to a 100% renewable power sector in 2050 in full hourly resolution and cost optimised 5-year intervals. The ratio of PV to wind started at 24% to 76%, respectively, and gradually changed its global average complementarity to 79% to 21%, respectively. One reason is the stronger demand growth in Sun Belt countries, but that fundamental trend can be also found in very well developed regions, as confirmed also on a more regional level for the major region of North America, where the PV to wind ratio develops from 16% to 84% in 2015 to 62% to 38% in 2050, respectively.
The two cases for cross-border grid interconnection and relative cost changes during the transition period highlight that the underlying meteorological wind-solar complementarity is also affected by economic constraints for cost-optimised system solutions, as required by policymakers and energy system planners.
While the preceding discussion clarifies the benefit of complementarity, it was not possible to find a technique that can ease the implementation of some complementarity criteria during system design. Most economic studies report what they find as an economically optimal option according to given constraints, thus lack a means to fully enforce complementarity particularly in a way that values its contribution to improve ramping need and reliability. This is with the consideration of modern energy system analyses done on an hourly scale by including gas turbines, engines, and batteries for fast ramping and balancing, while electrolysers, smart charging and vehicle-to-grid can further balance power systems with very fast response and high accuracy. The challenge of the existing models is due to the complexity of the tools that measure some of these benefits, which require considering wide-ranging design criteria [6,19] and a better standard. The weakness in the existing methods and indicators [49], particularly the absence of a straight forward parameter that can measure specific characteristics, presents a major challenge to ascribe a specific value as to its benefit to the power system during the system design. At the same time, local conditions can limit the benefit of this complementarity because, among other things, the matching of resources to local demand should be optimised in a way that would ensure continuous reliability and security. Thus, further studies are necessary to effectively exploit this potential.
We evaluated the benefits of the complementarity of resources based on the data presented in literature. These sources show that: (1) without energy storage, wind-solar complementarity leads to a significantly higher penetration of VRE as compared to both resources as a stand-alone. At these stages and considering only technical, but not economic constraints, reaching high penetration appears to favour a higher wind energy contribution than solar due to its ability to generate electricity at night; (2) wind-solar complementarity leads to significantly higher penetration of VRE at significantly reduced energy storage and balancing capacities. When curtailment is allowed, wind-solar complementarity gives a significant advantage by further reducing the storage and balancing capacity required to reach a certain penetration level. In the presence of storage to arrive at higher penetration, the solar share appears to dominate because of its well-defined periodic output that allows storing during day and discharging at night. This effect reduces storage capacity and the need for more wind energy generation outside the solar generation window; (3) wind-solar complementarity was also linked to reduced ramping need and improved reliability of energy supply. However, tools that can ease consideration of complementarity during system design have not been found in the literature.
Achieving a broader complementarity by incorporating other RE resources could lead to a 100% renewable energy system with much lower energy storage. Some studies have already reported a 100% renewable power system without the need for significant storage through resource complementarity as compared to those which rely on less diverse resources and need larger storage.
The authors gratefully acknowledge the public financing of Tekes, the Finnish Funding Agency for Innovation, for the ‘Neo-Carbon Energy’ project under the number 40101/14 and support from LUT internally funded research platform REFLEX. UC would like to thank Reiner Lemoine-Foundation for the valuable scholarship. SAA would like to acknowledge partial funding from Academy of Finland, for investigating biophysical limits of energy transition (317681).
The authors declare no conflict of interest.
[1] |
Solomon AA (2019) Large scale photovoltaics and the future energy system requirement. AIMS Energy 7: 600-618. doi: 10.3934/energy.2019.5.600
![]() |
[2] |
Denholm P, Margolis RM (2007) Evaluating the limits of solar photovoltaics (PV) in electric power systems utilizing energy storage and other enabling technologies. Energy Policy 35: 4424-4433. doi: 10.1016/j.enpol.2007.03.004
![]() |
[3] |
Lund PD, Lindgren J, Mikkola J, et al. (2015) Review of energy system flexibility measures to enable high levels of variable renewable electricity. Renewable Sustainable Energy Rev 45: 785-807. doi: 10.1016/j.rser.2015.01.057
![]() |
[4] |
Konziella H, Bruckner T (2016) Flexibility requirements of renewable energy based electricity systems-a review of research results and methodologies. Renewable Sustainable Energy Rev 53: 10-22. doi: 10.1016/j.rser.2015.07.199
![]() |
[5] |
Heide DM, Greiner Bremen L, Hoffmann C (2011) Reduced storage and balancing needs in a fully renewable European power system with excess wind and solar power generation. Renewable Energy 36: 2515-2523. doi: 10.1016/j.renene.2011.02.009
![]() |
[6] |
Solomon AA, Kammen DM, Callaway D (2016) Investigating the impact of wind-solar complementarities on energy storage requirement and the corresponding supply reliability criteria. Appl Energ 168: 130-145. doi: 10.1016/j.apenergy.2016.01.070
![]() |
[7] |
Heide D, Bremen L, Greiner M, et al. (2010) Seasonal optimal mix of wind and solar power in a future, highly renewable Europe. Renewable Energy 35: 2483-2489. doi: 10.1016/j.renene.2010.03.012
![]() |
[8] |
Esteban M, Zhang Q, Utama A (2012) Estimation of the energy storage requirement of a future 100% renewable energy system in Japan. Energy Policy 47: 22-31. doi: 10.1016/j.enpol.2012.03.078
![]() |
[9] | Budischak C, Sewell D, Thomson H, et al. (2013) Cost-minimized combinations of wind power, solar power and electrochemical storage, powering the grid up to 99.9% of the time. J Power Sources 225: 60-74. |
[10] |
Child M, Breyer C (2016) The role of energy storage solutions in a 100% renewable Finnish energy system. Energy Procedia 99: 25-34. doi: 10.1016/j.egypro.2016.10.094
![]() |
[11] | Caldera U, Bogdanov D, Afanasyeva S, et al. (2016) Integration of reverse osmosis seawater desalination in the power sector, based on PV and wind energy, for the Kingdom of Saudi Arabia. Proceedings of 32nd European Photovoltaic Solar Energy Conference, Munchen, 21-24. |
[12] |
Bogdanov D, Breyer C (2016) North-East Asian Super Grid for 100% renewable energy supply: Optimal mix of energy technologies for electricity, gas and heat supply options. Energ Convers Manage 112: 176-190. doi: 10.1016/j.enconman.2016.01.019
![]() |
[13] |
Child M, Breyer C (2016) Vision and initial feasibility analysis of a recarbonised Finnish energy system. Renewable Sustainable Energy Rev 66: 517-536. doi: 10.1016/j.rser.2016.07.001
![]() |
[14] |
Babrowski S, Jochem P, Fichtner W (2016) Electricity storage systems in the future German energy sector-An optimization of the German electricity generation system until 2040 considering grid restrictions. Comput Oper Res 66: 228-240. doi: 10.1016/j.cor.2015.01.014
![]() |
[15] |
Mileva A, Nelson JH, Johnston J, et al. (2013) Sunshot solar power reduces costs and uncertainty in future low-carbon electricity systems. Environ Sci Technol 47: 9053-9060. doi: 10.1021/es401898f
![]() |
[16] |
Brouwer A, van den Broek M, Zappa W, et al. (2016) Least-cost options for integrating intermittent renewables in low-carbon power systems. Appl Energ 161: 48-74. doi: 10.1016/j.apenergy.2015.09.090
![]() |
[17] |
Solomon AA, Faiman D, Meron G (2010) Properties and uses of storage for enhancing the grid penetration of very large-scale photovoltaic systems. Energy Policy 38: 5208-5222. doi: 10.1016/j.enpol.2010.05.006
![]() |
[18] | Solomon AA, Faiman D, Meron G (2011) Appropriate storage for high-penetration grid-connected photovoltaic plants. Energy Policy 40: 335-344. |
[19] |
Solomon AA, Kammen DM, Callaway D (2014) The role of large-scale energy storage design and dispatch in the power grid: a study of very high grid penetration of variable renewable resources. Appl Energ 134: 75-89. doi: 10.1016/j.apenergy.2014.07.095
![]() |
[20] |
Solomon AA, Faiman D, Meron G (2010) Grid matching of large-scale wind energy conversion systems, alone and in tandem with large-scale photovoltaic systems: An Israeli case study. Energy Policy 38: 7070-7081. doi: 10.1016/j.enpol.2010.07.026
![]() |
[21] |
Solomon AA, Faiman D, Meron G (2010) The effects on grid matching and ramping requirements, of single and distributed PV systems employing various fixed and sun-tracking technologies. Energy Policy 38: 5469-5481. doi: 10.1016/j.enpol.2010.02.056
![]() |
[22] |
Solomon AA, Faiman D, Meron G (2010) An energy-based evaluation of the matching possibilities of very large photovoltaic plants to the electricity grid: Israel as a case study. Energy Policy 38: 5457-5468. doi: 10.1016/j.enpol.2009.12.024
![]() |
[23] |
Kroposki B (2017) Integrating high level of variable renewable energy into electricity power systems. J Mod Power Syst Cle 5: 831-827. doi: 10.1007/s40565-017-0339-3
![]() |
[24] |
Solomon AA, Bogdanov D, Breyer C (2019) Curtailment-storage-penetration nexus in energy transition. Appl Energ 235: 1351-1368. doi: 10.1016/j.apenergy.2018.11.069
![]() |
[25] |
Miglietta MM, Huld T, Monforti-Ferrario F (2017) Local complementarity of wind and solar energy resources over Europe: An assessment study from a meteorological perspective. J Appl Meteorol Climatol 56: 217-234. doi: 10.1175/JAMC-D-16-0031.1
![]() |
[26] | Monferti F, Huld T, Bódis K, et al. (2014) Assessing complementarity of wind and solar resources for energy production in Italy. A Monte Carlo approach. Renewable Energy 63: 576-586. |
[27] |
Hoicha, EC, Rowlands IH (2011) Solar and wind resource complementarity: Advancing options for renewable electricity integration in Ontario, Canada. Renewable Energy 36: 97-107. doi: 10.1016/j.renene.2010.06.004
![]() |
[28] | Gerlach AK, Stetter D, Schmid J, et al. (2011) PV and wind power-complementary technologies. 26th European Photovoltaic Solar Energy Conference, Hamburg, 5-9. |
[29] |
Huber M, Dimkova D, Hamacher T (2014) Integration of wind and solar power in Europe: Assessment of flexibility requirements. Energy 69: 236-246. doi: 10.1016/j.energy.2014.02.109
![]() |
[30] |
Rosa CDOCS, Costa KA, Christo EDS, et al. (2017) Complementarity of hydro, photovoltaic, and wind power in Rio de Janeiro State. Sustainability 9: 1130. doi: 10.3390/su9071130
![]() |
[31] |
Lund H (2006) Large-scale integration of optimal combinations of PV, wind and wave power into the electricity supply. Renewable Energy 31: 503-515. doi: 10.1016/j.renene.2005.04.008
![]() |
[32] |
Slusarewicz JH, Cohan DS (2018) Assessing solar and wind complementarity in Texas. Renew Wind Water Solar 5: 7. doi: 10.1186/s40807-018-0054-3
![]() |
[33] | da Luz T, Moura P (2019) Power generation expansion planning with complementarity between renewable sources and regions for 100% renewable energy systems. Int T Electr Energy 29: e2817. |
[34] |
Shaner MR, Davis SJ, Lewis NS, et al. (2018) Geophysical constraints on the reliability of solar and wind power in the United States. Energy Environ Sci 11: 914-925. doi: 10.1039/C7EE03029K
![]() |
[35] | Huang B, Krishnan V, Hodge BM (2018) Analyzing the impacts of variable renewable resources on California net-load ramp events. 2018 IEEE Power & Energy Society General Meeting, Portland, 5-10. |
[36] |
Risso A, Beluco A, Alves RCM (2018) Complementarity roses evaluating spatial complementarity in time between energy resources. Energies 11: 1918. doi: 10.3390/en11071918
![]() |
[37] |
Min CG, Kim MK (2017) Impact of the complementarity between variable generation resources and load on the flexibility of the Korean power system. Energies 10: 1719. doi: 10.3390/en10111719
![]() |
[38] |
Santos-Alamillos FJ, Pozo-Vazques D, Ruiz-Arias JA, et al. (2015) Combining wind farms with concentrating solar plants to provide stable renewable power. Renewable Energy 76: 539-550. doi: 10.1016/j.renene.2014.11.055
![]() |
[39] |
Gallardo RP, Rios AM, Ramirez JS (2020) Analysis of the solar and wind energetic complementarity in Mexico. J Clean Prod 268: 122323. doi: 10.1016/j.jclepro.2020.122323
![]() |
[40] |
Han S, Zhang L, Liu Y, et al. (2019) Quantitative evaluation method for the complementarity of wind-solar-hydro power and optimization of wind-solar ratio. Appl Energ 236: 973-984. doi: 10.1016/j.apenergy.2018.12.059
![]() |
[41] | Sterl S, Vanderkelen I, Chawanda CJ, et al. (2020) Smart renewable electricity portfolios in West Africa. Nat Sustain |
[42] |
Oyewo AS, Aghahosseini A, Ram M, et al. (2020) Transition towards decarbonized power systems and its socio-economic impacts in West Africa. Renewable Energy 154: 1092-1112. doi: 10.1016/j.renene.2020.03.085
![]() |
[43] |
Jurasz J, Beluco A, Canales FA (2018) The impact of complementarity on power supply reliability of small scale hybrid energy systems. Energy 161: 737-743. doi: 10.1016/j.energy.2018.07.182
![]() |
[44] |
Demissie AA, Solomon AA (2016) Power system sensitivity to extreme hydrological conditions as studied using an integrated reservoir and power system dispatch model, the case of Ethiopia. Appl Energ 182: 442-463. doi: 10.1016/j.apenergy.2016.08.106
![]() |
[45] |
Barbosa LDSNS, Orozco JF, Bogdanov D, et al. (2016) Hydropower and power-to-gas storage options: The Brazilian energy system case. Energy Procedia 99: 89-107. doi: 10.1016/j.egypro.2016.10.101
![]() |
[46] |
Solomon AA, Child M, Caldera U, et al. (2017) How much energy storage is needed to incorporate very large intermittent renewables? Energy Procedia 135: 283-293. doi: 10.1016/j.egypro.2017.09.520
![]() |
[47] |
Pensini A, Rasmussen CN, Kempton W (2014) Economic analysis of using excess renewable electricity to displace heating fuels. Appl Energ 131: 530-543. doi: 10.1016/j.apenergy.2014.04.111
![]() |
[48] | Mensah TNO The role of modern bioenergy in solar photovoltaic driven and defossilised power systems-The case of Ghana. MSc Thesis, LUT University, Lappeenranta, 2019 |
[49] |
Weschenfelder F, Leite GNP, Costa AC, et al. (2020) A review on the complementarity between grid-connected solar and wind power systems. J Clean Prod 257: 120617. doi: 10.1016/j.jclepro.2020.120617
![]() |
[50] |
Jurasz J, Canales FA, Kies A, et al. (2020) A review on the complementarity of renewable energy sources: Concept, metrics, application and future research directions. Sol Energy 195: 703-724. doi: 10.1016/j.solener.2019.11.087
![]() |
[51] | Yan J, Qu T, Han S, et al. (2020) Reviews on characteristic of renewables: Evaluating the variability and complementarity. Int T Electr Energy 30: e12281. |
[52] |
Liu LJ, Wang Z, Wang Y, et al. (2020) Optimizing wind/solar combinations at finer scales to mitigate renewable energy variability in China. Renewable Sustainable Energy Rev 132: 110151. doi: 10.1016/j.rser.2020.110151
![]() |
[53] |
Xu L, Wang ZW, Liu YF (2017) The spatial and temporal variation features of wind-sun complementarity in China. Energ Convers Manage 154: 138-148. doi: 10.1016/j.enconman.2017.10.031
![]() |
[54] |
Zhang HX, Cao YJ, Zhang Y, et al. (2018) Quantitative synergy assessment of regional wind-solar energy resources based on MERRA reanalysis data. Appl Energ 216: 172-182. doi: 10.1016/j.apenergy.2018.02.094
![]() |
[55] | Holttinen H, Kiviluoma J, Levy T, et al. (2016) Design and Operation of Power Systems with Large Amounts of Wind Power: Final Summary Report, IEA WIND Task 25. Available from: https://www.vttresearch.com/sites/default/files/pdf/technology/2016/T268.pdf. |
[56] |
Child M, Kemfert C, Bogdanov D, et al. (2019) Flexible electricity generation, grid exchange and storage for the transition to a 100% renewable energy system in Europe. Renewable Energy 139: 80-101. doi: 10.1016/j.renene.2019.02.077
![]() |
[57] | Cui M, Zhang J, Florita AR, et al. (2015) An Optimized swinging door algorithm for wind power ramp event detection. 2015 IEEE Power & Energy Society General Meeting, 26-30. |
[58] |
Debnath K, Goel L (1995) Power system planning-a reliability perspective. Electr Pow Syst Res 34: 179-185. doi: 10.1016/0378-7796(95)00976-X
![]() |
[59] | Breyer C, Bogdanov D, Aghahosseini A, et al. (2020) On the techno-economic benefits of a global energy interconnection. EEEP 9: 83-102. |
[60] |
Bogdanov D, Farfan J, Sadovskaia K, et al. (2019) Radical transformation pathway towards sustainable electricity via evolutionary steps. Nat Commun 10: 1077. doi: 10.1038/s41467-019-08855-1
![]() |
[61] | Ram M, Bogdanov D, Aghahosseini A, et al. (2019) Global Energy System Based on 100% Renewable Energy-Power, Heat, Transport and Desalination Sectors, Lappeenranta: LUT University and Energy Watch Group. |
[62] | Ram M, Bogdanov D, Aghahosseini A, et al. (2020) 100% Renewable Europe: How to Make Europe's Energy System Climate-Neutral Before 2050, Brussels and Lappeenranta: SolarPower Europe and LUT University. |
[63] |
Caldera U, Bogdanov D, Breyer C (2016) Local cost of seawater RO desalination based on solar PV and wind energy-A global estimate. Desalination 385: 207-216. doi: 10.1016/j.desal.2016.02.004
![]() |
[64] |
Breyer C, Bogdanov D, Gulagi A, et al. (2017) On the role of solar photovoltaics in global energy transition scenarios. Prog Photovolt 25: 727-745. doi: 10.1002/pip.2885
![]() |
[65] |
Soder L, Tomasson E, Estanqueiro A, et al. (2020) Review of wind generation within adequacy calculations and capacity markets for different power systems. Renewable Sustainable Energy Rev 119: 109540. doi: 10.1016/j.rser.2019.109540
![]() |
[66] |
Caldera U, Breyer C (2018) The role that battery and water storage play in Saudi Arabia's transition to an integrated 100% renewable energy power system. J Energy Storage 17: 299-310. doi: 10.1016/j.est.2018.03.009
![]() |
[67] |
Azzuni A, Breyer C (2020) Global Energy Security index and its Application on National level. Energies 13: 2502. doi: 10.3390/en13102502
![]() |
[68] |
Breyer C, Tsupari E, Tikka V, et al. (2015) Power-to-gas as an emerging profitable business through creating an integrated value chain. Energy Procedia 73: 182-189. doi: 10.1016/j.egypro.2015.07.668
![]() |
[69] |
Child M, Nordling A, Breyer C (2018) The impacts of high V2G participation in a 100% renewable Åland energy system. Energies 11: 2206. doi: 10.3390/en11092206
![]() |
[70] | Taljegard M, Walter V, Goransson L, et al. (2019) Impact of electric vehicles on the cost-competitiveness of generation and storage technologies in electricity system. Environ Res Lett 14: 12. |
1. | David Ludwig, Christian Breyer, A. A. Solomon, Robert Seguin, Evaluation of an onsite integrated hybrid PV-Wind power plant, 2020, 8, 2333-8334, 988, 10.3934/energy.2020.5.988 | |
2. | Jakub Jurasz, Jerzy Mikulik, Paweł B. Dąbek, Mohammed Guezgouz, Bartosz Kaźmierczak, Complementarity and ‘Resource Droughts’ of Solar and Wind Energy in Poland: An ERA5-Based Analysis, 2021, 14, 1996-1073, 1118, 10.3390/en14041118 | |
3. | Mohammed Guezgouz, Jakub Jurasz, Mohamed Chouai, Hannah Bloomfield, Benaissa Bekkouche, Assessment of solar and wind energy complementarity in Algeria, 2021, 238, 01968904, 114170, 10.1016/j.enconman.2021.114170 | |
4. | M. K. Islam, N. M. S. Hassan, M. G. Rasul, Kianoush Emami, Ashfaque Ahmed Chowdhury, Green and renewable resources: an assessment of sustainable energy solution for Far North Queensland, Australia, 2022, 2008-9163, 10.1007/s40095-022-00552-y | |
5. | Oskar Lindberg, Johan Arnqvist, Joakim Munkhammar, David Lingfors, Review on power-production modeling of hybrid wind and PV power parks, 2021, 13, 1941-7012, 042702, 10.1063/5.0056201 | |
6. | Diana Cantor, Andrés Ochoa, Oscar Mesa, Total Variation-Based Metrics for Assessing Complementarity in Energy Resources Time Series, 2022, 14, 2071-1050, 8514, 10.3390/su14148514 | |
7. | Imre M. Jánosi, Karim Medjdoub, Miklós Vincze, Combined wind-solar electricity production potential over north-western Africa, 2021, 151, 13640321, 111558, 10.1016/j.rser.2021.111558 | |
8. | Karl Ezra S. Pilario, Jessa A. Ibañez, Xaviery N. Penisa, Johndel B. Obra, Carl Michael F. Odulio, Joey D. Ocon, Spatio-Temporal Solar–Wind Complementarity Assessment in the Province of Kalinga-Apayao, Philippines Using Canonical Correlation Analysis, 2022, 14, 2071-1050, 3253, 10.3390/su14063253 | |
9. | Rebecca Peters, Jürgen Berlekamp, Klement Tockner, Christiane Zarfl, RePP Africa – a georeferenced and curated database on existing and proposed wind, solar, and hydropower plants, 2023, 10, 2052-4463, 10.1038/s41597-022-01922-1 | |
10. | Richard Morse, Sarah Salvatore, Joanna H. Slusarewicz, Daniel S. Cohan, Can wind and solar replace coal in Texas?, 2022, 9, 2198-994X, 10.1186/s40807-022-00069-2 | |
11. | Constantino Dário Justo, José Eduardo Tafula, Pedro Moura, Planning Sustainable Energy Systems in the Southern African Development Community: A Review of Power Systems Planning Approaches, 2022, 15, 1996-1073, 7860, 10.3390/en15217860 | |
12. | Jakub Jurasz, Mohammed Guezgouz, 2022, 9780323855273, 359, 10.1016/B978-0-323-85527-3.00024-8 | |
13. | Arman Aghahosseini, A.A. Solomon, Christian Breyer, Thomas Pregger, Sonja Simon, Peter Strachan, Arnulf Jäger-Waldau, Energy system transition pathways to meet the global electricity demand for ambitious climate targets and cost competitiveness, 2023, 331, 03062619, 120401, 10.1016/j.apenergy.2022.120401 | |
14. | Javier López Prol, Fernando deLlano-Paz, Anxo Calvo-Silvosa, Stefan Pfenninger, Iain Staffell, Spatial integration for firm and load-following wind generation, 2024, 19, 1748-9326, 094026, 10.1088/1748-9326/ad5d7d | |
15. | Hasret Sahin, Abebe Asfaw Solomon, Arman Aghahosseini, Christian Breyer, The impact of spatial representation in energy transition modelling on systemwide energy return on investment, 2024, 18, 1752-1416, 2706, 10.1049/rpg2.13117 | |
16. | Theophilus Nii Odai Mensah, Ayobami Solomon Oyewo, Dmitrii Bogdanov, Arman Aghahosseini, Christian Breyer, Pathway for a fully renewable power sector of Africa by 2050: Emphasising on flexible generation from biomass, 2024, 234, 09601481, 121198, 10.1016/j.renene.2024.121198 | |
17. | A.A. Solomon, Nelson Bunyui Manjong, Christian Breyer, The necessity to standardise primary energy quality in achieving a meaningful quantification of related indicators, 2023, 12, 26669552, 100115, 10.1016/j.segy.2023.100115 | |
18. | Galina Deryugina, Evgeny Ignatev, Htet Myat Htun, 2023, Determination of the Optimal Configuration of Solar PV Power Plants and Wind Farms in the United Energy System of Myanmar, 979-8-3503-0339-1, 117, 10.1109/UralCon59258.2023.10291143 | |
19. | Arben Gjukaj, Rexhep Shaqiri, Qamil Kabashi, Vezir Rexhepi, Renewable energy integration and distributed generation in Kosovo: Challenges and solutions for enhanced energy quality, 2024, 12, 2333-8334, 686, 10.3934/energy.2024032 | |
20. | Sonia Jerez, David Barriopedro, Alejandro García‐López, Raquel Lorente‐Plazas, Andrés M. Somoza, Marco Turco, Judit Carrillo, Ricardo M. Trigo, An Action‐Oriented Approach to Make the Most of the Wind and Solar Power Complementarity, 2023, 11, 2328-4277, 10.1029/2022EF003332 | |
21. | Rebecca Peters, Jürgen Berlekamp, Charles Kabiri, Beth A. Kaplin, Klement Tockner, Christiane Zarfl, Sustainable pathways towards universal renewable electricity access in Africa, 2024, 5, 2662-138X, 137, 10.1038/s43017-023-00501-1 | |
22. | Raheel A. Shaikh, David J. Vowles, Andrew Allison, Derek Abbott, Evaluation of Australia’s Generation-Storage Requirements in a Fully Renewable Grid With Intermittent and Flexible Generation, 2023, 11, 2169-3536, 64201, 10.1109/ACCESS.2023.3286037 | |
23. | Oscar Delbeke, Jens D. Moschner, Johan Driesen, The complementarity of offshore wind and floating photovoltaics in the Belgian North Sea, an analysis up to 2100, 2023, 218, 09601481, 119253, 10.1016/j.renene.2023.119253 | |
24. | Øyvind Sommer Klyve, Ville Olkkonen, Magnus Moe Nygård, David Lingfors, Erik Stensrud Marstein, Oskar Lindberg, Retrofitting wind power plants into hybrid PV–wind power plants: Impact of resource related characteristics on techno-economic feasibility, 2025, 379, 03062619, 124895, 10.1016/j.apenergy.2024.124895 | |
25. | Russell McKenna, Johan Lilliestam, Heidi U. Heinrichs, Jann Weinand, Johannes Schmidt, Iain Staffell, Andrea N. Hahmann, Peter Burgherr, Arne Burdack, Monika Bucha, Ruihong Chen, Michael Klingler, Paul Lehmann, Jens Lowitzsch, Riccardo Novo, James Price, Romain Sacchi, Patrick Scherhaufer, Eva M. Schöll, Piero Visconti, Paola Velasco-Herrejón, Marianne Zeyringer, Luis Ramirez Camargo, System impacts of wind energy developments: Key research challenges and opportunities, 2025, 9, 25424351, 101799, 10.1016/j.joule.2024.11.016 | |
26. | Jialin Song, Haoyi Zhang, Yanming Zhang, Zhongjiao Ma, Mingfei He, Research progress on industrial waste heat recycling and seasonal energy storage, 2025, 13, 2333-8334, 147, 10.3934/energy.2025006 | |
27. | Katarina Obradović, Nouha Dkhili, Moataz El Sied, Pierre-Elouan Mikael Réthoré, Kaushik Das, From the idea to construction: Aspects of relevance of the optimized physical design of renewable hybrid power plants, 2025, 78, 22131388, 104325, 10.1016/j.seta.2025.104325 |
Country/region | Share of solar technology in the total VRE capacity | |||
0 | 0.5 | 1 | ||
Penetration (% of annual demand) | Israel (5% curtailment) | 32 | 40* | 29 |
Israel (0% curtailment) | 17 | 19 | 17 | |
California (~0% curtailment) | 26 | 36+ | 27 | |
California (~5% curtailment) | 40 | 52 | 41 | |
+: The optimal mix gives approximately 38.2%; *: Penetration reaches 46% for concentrating photovoltaic (CPV) technologies. |
Solar share (% total generation) | Penetration (% annual demand) | Curtailment (% generation) | Remark |
0 | ~75 | ~25 | Though the 25% wind share achieves approximately the same penetration at equal curtailment as compared to the 100% wind system, note that it required lesser areas of aggregation. For the same aggregation area, Figure 2 suggests that penetration and curtailment corresponding to a 100% wind energy generation mix is approximately 70% and 30%, respectively. |
25 | 75 | 25 | |
50 | 73 | 27 | |
75 | 60 | 40 | |
100 | 50 | 50 |
Total energy loss (% of VRE generation) | Parameters | Solar share of total VRE by capacity | Storage energy capacity | ||
0% | 50% | 100% | |||
0% | VRE penetration (% of annual demand) | 38 | 58 | 52 | 300 GWh |
Required balancing capacity (GW) | 56 | 47 | 46 | 300 GWh | |
15% | VRE penetration (% of annual demand) | 55 | 81 | 62.9 | 186 GWh |
Required balancing capacity (GW) | 52 | 31 | 39 | 186 GWh |
Total VRE capacity (GW) | Penetration (% of annual demand) | Network energy capacity (GWh) | Network power capacity (GW) | Balancing capacity (GW) | Wind (% of total VRE capacity) |
65.5 | 52.6 | 4730.6 | 44.4 | 50.3 | 100 |
58.2 | 51.9 | 424.4 | 35.8 | 50.3 | 75 |
58.2 | 52.1 | 320.2 | 22.2 | 50.7 | 70 |
58.2 | 52.2 | 232.2 | 20.6 | 51.3 | 65 |
58.2 | 52.3 | 201.3 | 21.7 | 51.4 | 60 |
58.2 | 52.3 | 165.9 | 19.4 | 51.5 | 55 |
58.2 | 52.2 | 154.2 | 18.1 | 51.7 | 50 |
58.2 | 52.1 | 153.8 | 18.4 | 51.3 | 45 |
58.2 | 51.8 | 160.6 | 18.7 | 51.2 | 40 |
67.6 | 51.9 | 322.3 | 29.0 | 48.3 | 0 |
Parameters of interest | Region/country of the study | ||||||
Finland integrated [10] | KSAa integrated [11] | KSAa [11] | Israel [17] | California [19] | Europe [5] | PJMb [9,47] | |
VRE penetration (% of annual demand) | 70 | 99 | 98 | 90 | 85 | 100 | 100 |
Energy storage capacity (GWh) | 3990 | 43407 | 45398 | 113 | 186 | 16,000 | 891 |
Energy storage capacity (daily average demand) | 8.6 | 16.5 | 19.4 | 0.83 | 0.22 | 1.8 | 1.2 |
Total energy loss (% of total VRE generation) | 6 (2.5% storage loss) | 13.4 (9% storage loss) | 14.6 (10% storage loss) | 20 (12% storage loss) | 20 (3% storage loss) | 50c | > 50c, d |
Annual demand during the studied year (TWh) | 105 | 729 | 650 | 50.2 | 302 | 3240 | 276 |
Usefulness index (a.u.) | 6.3e | 11.6e | 10e | 186 | 220 | NAf | 24 |
Storage efficiency | mixg | mixg | mixg | 75% | 75% | 100% | 81% |
Share of other RE resources (% of annual demand) | 30 (hydro, biomass) | 1 (geothermal) | 2 (geothermal) | 10h | 15h | 0 | 0 |
Wind share (% of total VRE by capacity) | 54 | 39 | 40 | 0 | 43 | 60 | 93 |
Energy sectors investigated | power, thermal sector | power, desalination | power | power | power | power | power |
a: Data correspond to the result of year 2050; b: This is based on the GIV storage scenario, which was selected for data completeness; c: Estimate may not include loss due to storage efficiency; d: Estimate based on the given data [9], in [47] it was shown that at 95% penetration the loss was 51%; e: UI could be lower for low efficiency storage because of the resulting larger energy storage need, UI for KSA was readjusted for gas storage (the improvement in UI was to maximum 5 points). For Finland, direct use of synthetic gas makes it less important (but even if necessary, the change will be a maximum 5 points); f: At 50% curtailment, for a diverse resource such as theirs, a penetration of approximately 80% is possible, one could then see the UI will at maximum be 40.5; g: Resource type not specified, but considered dispatchable renewable technologies (at the same time, data also show 98% VRE penetration at 25% total energy loss as well as storage capacity of about 1.3 and 6 times the daily average demand of California and Israel, respectively; h: The dominant capacities and their efficiencies are power-to-gas (electrolyser, 61% [10], 77% [11], CCGT 58% and OCGT 43%), battery (>90%), thermal energy storage = 90%. |
Country/region | Share of solar technology in the total VRE capacity | |||
0 | 0.5 | 1 | ||
Penetration (% of annual demand) | Israel (5% curtailment) | 32 | 40* | 29 |
Israel (0% curtailment) | 17 | 19 | 17 | |
California (~0% curtailment) | 26 | 36+ | 27 | |
California (~5% curtailment) | 40 | 52 | 41 | |
+: The optimal mix gives approximately 38.2%; *: Penetration reaches 46% for concentrating photovoltaic (CPV) technologies. |
Solar share (% total generation) | Penetration (% annual demand) | Curtailment (% generation) | Remark |
0 | ~75 | ~25 | Though the 25% wind share achieves approximately the same penetration at equal curtailment as compared to the 100% wind system, note that it required lesser areas of aggregation. For the same aggregation area, Figure 2 suggests that penetration and curtailment corresponding to a 100% wind energy generation mix is approximately 70% and 30%, respectively. |
25 | 75 | 25 | |
50 | 73 | 27 | |
75 | 60 | 40 | |
100 | 50 | 50 |
Total energy loss (% of VRE generation) | Parameters | Solar share of total VRE by capacity | Storage energy capacity | ||
0% | 50% | 100% | |||
0% | VRE penetration (% of annual demand) | 38 | 58 | 52 | 300 GWh |
Required balancing capacity (GW) | 56 | 47 | 46 | 300 GWh | |
15% | VRE penetration (% of annual demand) | 55 | 81 | 62.9 | 186 GWh |
Required balancing capacity (GW) | 52 | 31 | 39 | 186 GWh |
Total VRE capacity (GW) | Penetration (% of annual demand) | Network energy capacity (GWh) | Network power capacity (GW) | Balancing capacity (GW) | Wind (% of total VRE capacity) |
65.5 | 52.6 | 4730.6 | 44.4 | 50.3 | 100 |
58.2 | 51.9 | 424.4 | 35.8 | 50.3 | 75 |
58.2 | 52.1 | 320.2 | 22.2 | 50.7 | 70 |
58.2 | 52.2 | 232.2 | 20.6 | 51.3 | 65 |
58.2 | 52.3 | 201.3 | 21.7 | 51.4 | 60 |
58.2 | 52.3 | 165.9 | 19.4 | 51.5 | 55 |
58.2 | 52.2 | 154.2 | 18.1 | 51.7 | 50 |
58.2 | 52.1 | 153.8 | 18.4 | 51.3 | 45 |
58.2 | 51.8 | 160.6 | 18.7 | 51.2 | 40 |
67.6 | 51.9 | 322.3 | 29.0 | 48.3 | 0 |
Parameters of interest | Region/country of the study | ||||||
Finland integrated [10] | KSAa integrated [11] | KSAa [11] | Israel [17] | California [19] | Europe [5] | PJMb [9,47] | |
VRE penetration (% of annual demand) | 70 | 99 | 98 | 90 | 85 | 100 | 100 |
Energy storage capacity (GWh) | 3990 | 43407 | 45398 | 113 | 186 | 16,000 | 891 |
Energy storage capacity (daily average demand) | 8.6 | 16.5 | 19.4 | 0.83 | 0.22 | 1.8 | 1.2 |
Total energy loss (% of total VRE generation) | 6 (2.5% storage loss) | 13.4 (9% storage loss) | 14.6 (10% storage loss) | 20 (12% storage loss) | 20 (3% storage loss) | 50c | > 50c, d |
Annual demand during the studied year (TWh) | 105 | 729 | 650 | 50.2 | 302 | 3240 | 276 |
Usefulness index (a.u.) | 6.3e | 11.6e | 10e | 186 | 220 | NAf | 24 |
Storage efficiency | mixg | mixg | mixg | 75% | 75% | 100% | 81% |
Share of other RE resources (% of annual demand) | 30 (hydro, biomass) | 1 (geothermal) | 2 (geothermal) | 10h | 15h | 0 | 0 |
Wind share (% of total VRE by capacity) | 54 | 39 | 40 | 0 | 43 | 60 | 93 |
Energy sectors investigated | power, thermal sector | power, desalination | power | power | power | power | power |
a: Data correspond to the result of year 2050; b: This is based on the GIV storage scenario, which was selected for data completeness; c: Estimate may not include loss due to storage efficiency; d: Estimate based on the given data [9], in [47] it was shown that at 95% penetration the loss was 51%; e: UI could be lower for low efficiency storage because of the resulting larger energy storage need, UI for KSA was readjusted for gas storage (the improvement in UI was to maximum 5 points). For Finland, direct use of synthetic gas makes it less important (but even if necessary, the change will be a maximum 5 points); f: At 50% curtailment, for a diverse resource such as theirs, a penetration of approximately 80% is possible, one could then see the UI will at maximum be 40.5; g: Resource type not specified, but considered dispatchable renewable technologies (at the same time, data also show 98% VRE penetration at 25% total energy loss as well as storage capacity of about 1.3 and 6 times the daily average demand of California and Israel, respectively; h: The dominant capacities and their efficiencies are power-to-gas (electrolyser, 61% [10], 77% [11], CCGT 58% and OCGT 43%), battery (>90%), thermal energy storage = 90%. |