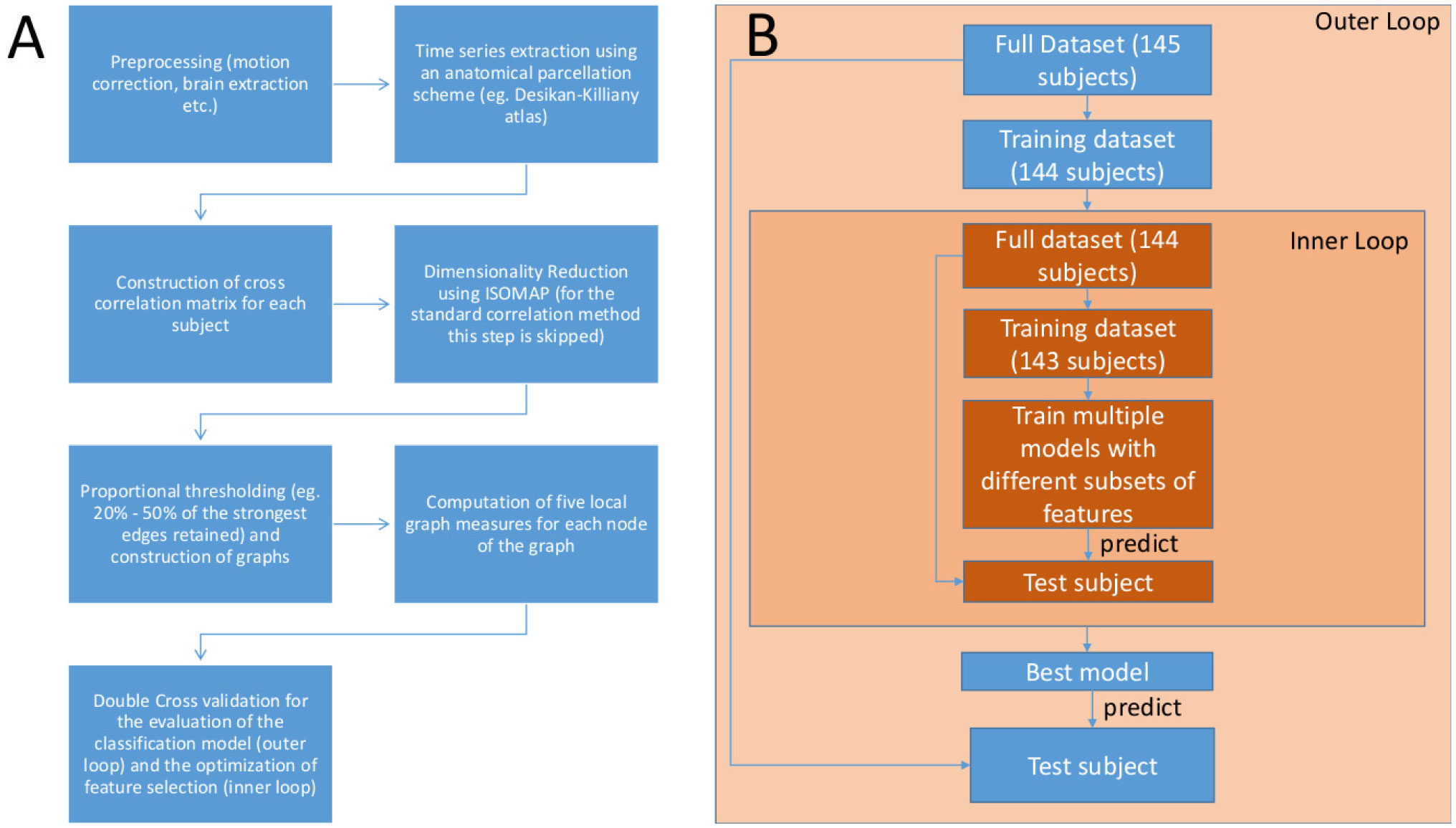
Citation: Chenwei Li, John A. Williams. Regulation of CCK-induced ERK1/2 activation by PKC epsilon in rat pancreatic acinar cells[J]. AIMS Molecular Science, 2017, 4(4): 463-477. doi: 10.3934/molsci.2017.4.463
[1] | Tien-Wen Lee, Gerald Tramontano . Automatic parcellation of resting-state cortical dynamics by iterative community detection and similarity measurements. AIMS Neuroscience, 2021, 8(4): 526-542. doi: 10.3934/Neuroscience.2021028 |
[2] | Evangelos Almpanis, Constantinos Siettos . Construction of functional brain connectivity networks from fMRI data with driving and modulatory inputs: an extended conditional Granger causality approach. AIMS Neuroscience, 2020, 7(2): 66-88. doi: 10.3934/Neuroscience.2020005 |
[3] | Rozaziana Ahmad, Khairunnuur Fairuz Azman, Rosliza Yahaya, Nazlahshaniza Shafin, Norsuhana Omar, Asma Hayati Ahmad, Rahimah Zakaria, Adi Wijaya, Zahiruddin Othman . Brain-derived neurotrophic factor (BDNF) in schizophrenia research: a quantitative review and future directions. AIMS Neuroscience, 2023, 10(1): 5-32. doi: 10.3934/Neuroscience.2023002 |
[4] | Aini Ismafairus Abd Hamid, Nurfaten Hamzah, Siti Mariam Roslan, Nur Alia Amalin Suhardi, Muhammad Riddha Abdul Rahman, Faiz Mustafar, Hazim Omar, Asma Hayati Ahmad, Elza Azri Othman, Ahmad Nazlim Yusoff . Distinct neural mechanisms of alpha binaural beats and white noise for cognitive enhancement in young adults. AIMS Neuroscience, 2025, 12(2): 147-179. doi: 10.3934/Neuroscience.2025010 |
[5] | Baptiste Lerosier, Gregory Simon, Sylvain Takerkart, Guillaume Auzias, Sonia Dollfus . Sulcal pits of the superior temporal sulcus in schizophrenia patients with auditory verbal hallucinations. AIMS Neuroscience, 2024, 11(1): 25-38. doi: 10.3934/Neuroscience.2024002 |
[6] | Ian M Rowbotham, Franco F Orsucci, Mohamed F Mansour, Samuel R Chamberlain, Haroon Y Raja . Relevance of Brain-derived Neurotrophic Factor Levels in Schizophrenia: A Systematic Review and Meta-Analysis. AIMS Neuroscience, 2015, 2(4): 280-293. doi: 10.3934/Neuroscience.2015.4.280 |
[7] | Fabrizio Turiaco, Fiammetta Iannuzzo, Giovanni Genovese, Clara Lombardo, Maria Catena Silvestri, Laura Celebre, Maria Rosaria Anna Muscatello, Antonio Bruno . Cognitive effects of brief and intensive neurofeedback treatment in schizophrenia: a single center pilot study. AIMS Neuroscience, 2024, 11(3): 341-351. doi: 10.3934/Neuroscience.2024021 |
[8] | Galina V. Portnova, Michael S. Atanov . Nonlinear EEG parameters of emotional perception in patients with moderate traumatic brain injury, coma, stroke and schizophrenia. AIMS Neuroscience, 2018, 5(4): 221-235. doi: 10.3934/Neuroscience.2018.4.221 |
[9] | Byron Bernal, Alfredo Ardila, Monica Rosselli . The Network of Brodmanns Area 22 in Lexico-semantic Processing: A Pooling-data Connectivity Study. AIMS Neuroscience, 2016, 3(3): 306-316. doi: 10.3934/Neuroscience.2016.3.306 |
[10] | Zarina Greenberg, Hayley Ramshaw, Quenten Schwarz . Time Windows of Interneuron Development: Implications to Our Understanding of the Aetiology and Treatment of Schizophrenia. AIMS Neuroscience, 2015, 2(4): 294-321. doi: 10.3934/Neuroscience.2015.4.294 |
Schizophrenia is a functional chronic mental disorder which affects how a person thinks, feels and acts. Symptoms may vary across patients and they include hallucinations, delusions, thought and movement disorders, disruptions in emotions and cognitive deficits. Prevalence of schizophrenia has reported to be around the 1% of the worldwide population without any significant difference between males and females [1]. In terms of brain connectivity, the “dysconnection hypothesis” has been the dominant theory on the cause of underlying brain malfunction in schizophrenia, suggesting both anatomical and functional brain dysfunction at different levels ranging from neurochemical to emerging functional connectivity impairments, thus demonstrating that schizophrenia could be perceived as a disorder of the human connectome [2]–[5].
Regarding the investigation of the functional brain connectome and neurological disorders, resting state magnetic resonance imaging (rsfMRI) has been proven a powerful tool [6]–[8]. This technique also holds the advantage of being non-invasive and at the same time capable of capturing deep structures of the brain (e.g. sub-cortical regions of the brain, such as the thalamus). Over the years, meta analysis of rsfMRI made possible the identification of the so-called resting state networks (RSN). These networks are reproducible across individual subjects and connect regions of the brain with certain functional properties [9]–[11]. Moreover, rsfMRI has also aided in both the detection of functional connectivity differences and the diagnosis of several neurological disorders, including schizophrenia [4], [12]–[14].
Until today, many studies have proposed several classification/diagnostic biomarkers of schizophrenia [15]–[19]. A typical pipeline for the construction of FCN includes a standard preprocessing routine (e.g. motion correction, removal of non-brain tissue etc.), the use of a parcellation scheme for signal extraction using e.g. Independent Component Analysis (ICA), or the extraction of time series directly in accordance to a predetermined anatomical or functional atlas, and finally the construction of FCN by cross-correlating the corresponding time series. Based on the constructed FCN, specific features are calculated and classifiers are implemented to classify between patients with schizophrenia and healthy controls. Typically, studies suggest the most informative features as biomarkers associated with the disease.
For example, for the construction of FCN and their classification, Cheng et al. [18] used correlation of rsfMRI time series from 278 anatomical atlas-derived nodes from a data set of 29 healthy and 19 schizophrenic subjects. Four different feature sets have been tested, namely, the betweenness centrality scores of all nodes, their ranks, the top ten highest scores and the top ten ranks. The best results were obtained using the rank of betweenness centrality of the top ten hubs of the network. They reported an accuracy of 79% using a single leave one-out cross-validation (LOOCV) and linear standard SVM (LSVM). Xiang et al. [15] also used correlation of time series extracted in accordance with a 246 node anatomical atlas from the COBRE dataset. They computed similar local graph measures to the ones computed here, namely, the degree of node, the participation coefficient, the local clustering coefficient, the betweenness centrality and the local efficiency. The authors utilized different feature selection algorithms such as the Least Absolute Shrinkage Operator (LASSO), Group LASSO (GLASSO) and Sparse Group LASSO (SGLASSO). Using LASSO and 123 features, they obtained a 83.4% classification, a 86.2% with GLASSO and 225 features, and a 93.1% with SGLASSO and 55 features. The evaluation method was one single LOOCV while the parameter tuning for each feature selection algorithm was done via grid search inside a single LOOCV. The classification algorithm that produced the best results in their study was the LSVM. Apparently, the authors, with SGLASSO, which is a two parameter feature selection method, optimized two parameters and trained the LSVM all within a single LOOCV scheme.
Eventually, as stated in [19], the validation method ( cross-validation) affects both the estimation of accuracy and the identification of the dominant features that could be represented as biomarkers. Indeed, Moghimi et al. [19] compared the results of single and double cross-validation schemes to a large dataset comprised of 170 subjects finding that a double cross-validation may lead to even a 20% decrease in the classification performance. The authors assessed more than 19000 global and local network measures and used the Sequential Feature Selection (SFS) algorithm in order to find the most predominant features, that could potentially serve as biomarkers. While using a single cross-validation, their best reported accuracy was 87% with 14 features, following a double cross-validation scheme their best reported accuracy was 73%, resulting from only a single feature. The addition of any other feature decreased the accuracy rate. They finally suggested that the cross-validation procedure may end up with inflated classification rates as a result of over-fitting to a specific dataset. The classification performance using single cross-validation analysis may be also apparently increased by utilizing more features that are less likely to generalize to independent datasets [19], [20].
In a recent study of Cai et al. [21], the authors applied a promising classification framework proposed in [22] to two independent datasets evaluating the within and between site generalizability of the model. The scope of the study was to test the generalizability of the current models for diagnosis and classification of schizophrenia. While with the proposed methodology, Du et al. [22] reported a 93% accuracy rate, the authors in [21] found a within-site accuracy rate of 73% and between-site accuracy of 70%. Finally, Cai et al. [21] attributed the findings to overfitting, a possible heterogeneity of their validating datasets and possible presence of noise, even if a comprehensive preprocessing routine had been applied. Interestingly, the approximately 20% difference in the expected accuracy was found also in the study of Moghimi et al. [19] between single and double cross-validation schemes.
Therefore, despite the fact that in some studies the reported classification performance is relatively high [15], [22], in general the obtained proposed accuracy rates and suggested biomarkers are diverse [19]–[21], [23]. There are several reasons why this happens. First of all, a single cross-validation procedure may end up with an over-optimistic estimate of model's performance [20], [24] especially when feature selection takes place [19]. Another reason is the small sample size [25], [26]. Also the pre-processing procedure may play an important role to the performance of the final classification model [19]. Thus, finding reliable/reproducible biomarkers (e.g. that generalize among different individuals) for schizophrenia remains a challenging problem [19], [21], [23], [25].
Besides the use of linear correlation for the construction of FCN, nonlinear manifold learning algorithms such as Isometric Mapping (ISOMAP) and Diffusion Maps, have been also applied for the construction of FCN [16], [27]. Other approaches such as cross-recurrence analysis and multilayer modelling [28] have been also proposed. Anderson and Cohen [16] used ISOMAP for the construction of embedded low-dimensional FCN for the classification between controls and schizophrenia patients using the COBRE dataset. ROIs were acquired using single subject ICA (for a review on ICA see in [29]). The analysis revealed differences in small-world properties among groups and 13 global-graph theoretic features led to a reported 65% accuracy rate (evaluating the model using a 10-fold cross validation scheme). Gallos et al. [27] used both linear (multidimensional scaling) and nonlinear manifold approaches (ISOMAP, Locally Linear Embedding, kernel PCA and Diffusion Maps) to construct embedded FCN based on the COBRE dataset. The classification performance of the global network properties was tested using key global graph-theoretic properties and several machine learning techniques including radial SVM and neural networks. The performance of two widely used metrics for the construction of FCN metrics, namely the Euclidean distance and the cross correlation metric was also assessed. As in [16] the analysis was performed based on the global graph theoretical measures.
Thus, here, building on recent methodological advances [16], [27], [30], we used ISOMAP to construct embedded FCN towards the classification of 71 schizophrenia patients and 74 healthy controls from the COBRE dataset, targeting at the local graph theoretical properties of the embedded FCN. Thus, we matched the different anatomical regions of each individual in accordance with the Desikan-Killiany Atlas [31]. This atlas has been recently used in a network-based analysis for exploring the dynamic functional core of human brain at a resting state [32]. We constructed FCN by (a) the standard approach, i.e. by-cross-correlating the rsfMRI time series [15], [18], [19] and, (b) using ISOMAP [16], [27], [33] to produce low dimensional embeddings of the correlation matrices. Based on the constructed FCN, we calculated five local graph theoretic measures, namely the participation coefficient, the strength of node, the betweenness centrality, the nodal efficiency and the local clustering coefficient. These measures have been well tried and tested in recent classification studies associated with schizophrenia [15], [18], [19], [34]. LASSO and Random Forest (RF) algorithms were used for feature selection. In order to select the most informative features and to evaluate the final model's performance we used a linear SVM with a double cross validation scheme.
The analysis is based on the Schizophrenia Centers of Biomedical Research Excellence (COBRE) dataset (publicly available at: http://fcon_1000.projects.nitrc.org/indi/retro/cobre.html). The COBRE dataset comprises of high resolution T1 images as well as rsfMRI data from 146 subjects. For the subject 0040075 there were missing rsfMRI data and subsequently it was excluded from further analysis, leaving a total of 71 Schizophrenic patients (Male/Female: 57/14; handedness R/L/B: 59/10/2; age: 38.1 ± 13.9) and 74 healthy controls (Male/Female: 51/23; handedness R/L/B: 71/1/2; age: 35.8 ± 11.5). All subjects were screened prior to any acquisition and exclusion criteria included: history of neurological disorder other than schizophrenia, mental retardation, severe head traumas with more than five minutes loss of consciousness, substance abuse or dependence within the last 12 months. Acquisition protocols were the same for both groups and included a T1-multi-echo-MPRAGE of high resolution (TR/TE/TI = 2530/[1.64, 3.5, 5.36, 7.22, 9.08]/900 ms, flip angle = 7°, matrix = 256×256×176, voxel-size = 1×1×1 mm3) and rs-fMRI with the single-shot echo planar imaging technique (TR: 2 s, TE: 29 ms, matrix size: 64×64, 32 slices, voxel-size: 3×3×4 mm3).
The fMRI data preprocessing was carried out using FEAT (FMRI Expert Analysis Tool) Version 6.00, part of FSL (FMRIB's Software Library). The pipeline included motion correction using Fsl's linear registration tools (MCFLIRT) [35], slice-timing correction using Fourier-space time-series phase-shifting, non-brain tissue removal using Fsl's brain extraction tool (BET) [36], spatial smoothing using a Gaussian kernel of 5mm Full Width at Half Maximum (FWHM), grand-mean intensity normalization of the entire 4D dataset by a single multiplicative factor. In order to further refine our data from noise due to motion artifacts, we also employed denoising via ICA AROMA methodology [37] which detects and regresses out noise-related independent components. High-pass filtering at 100Hz was applied after ICA AROMA procedure as it is recommended, in order to better identify motion-related components while at the same time avoiding ringing artifacts [37]. Additionally, a high-pass filter was favoured against a band-pass, as temporal band-pass filtering discards meaningful signal existing in higher frequencies [38].
Finally, FreeSurfer software package version 6.0.1 [39] was utilized to parcellate each individual T1-MPRAGE image into anatomical regions according to Desikan-Killiany (DK) Atlas [31]. Briefly, the FreeSurfer process pipeline included brain extraction, intensity normalization for the reconstruction of gray and white matter boundaries and pial surface extraction to approximately 150.000 vertices per hemisphere. For pairing each hemispheric surface with a spherical template of DK, atlas non-rigid transformations were performed and corrected iteratively until individual cortical folding patterns were matched with cortical geometry across subjects. Finally, parcellation of each individual subject was registered to the rsfMRI space and time series were extracted from 84 cortical and sub-cortical regions in a subject-specific manner. These time series were used for any further analysis.
For each pair of time series, here from M = 84 anatomical regions of the brain, say Ai and Aj, the cross-correlation function (CCF) (see also in [16]) reads:
where l represent the time lag (i.e. shifting in time, both backwards and forwards), and
For the construction of the FCN connectivity/distance matrices, we used a pseudo-distance metric dc defined as (see also in [16]):
However, the connectivity/distance matrices are hardly comparable across subjects, as they are fully connected [16]. Therefore, as a common practice, we set up a thresholding procedure to the (dis)similarity matrices in order to keep the strongest connections of the derived FCN. In order to remove the effect of the variable network density on the calculation of the graph measures across groups, we followed the approach of proportional thresholding (PT) [41]. We examined different levels of PT ranging from 20% to 50% (similar ranges have been also used in recent studies [4], [15], [18]) with a step of 5%. Other approaches of thresholding that have been proposed include data-driven topological filtering based on Orthogonal Minimum Spanning Trees (OMST) with applications in different neuroimaging modalities [42], [43].
ISOMAP is a non-linear dimensionality reduction/manifold learning algorithm that considering a set of M objects/observables
where
Here, the observables xi are the amplitudes of time series matched to anatomical regions of the brain
The steps of the algorithm that solves the above minimization problem can be described briefly as follows [33]:
1. Create a graph
2. Calculate the shortest paths (geodesic distances)
3. Estimate the new coordinates of the low-dimensional embedded manifold
For the new embedded coordinates
The selection of the embedding dimension p was based on the spectrum of the eigenvalues resulting from the last step of the algorithm (the MDS decomposition on the geodesic distance matrix DG) (see also in
We assessed five key local graph measures as described in
1. Strength of node:
2. Local efficiency:
3. Local clustering coefficient:
4. Betweenness centrality:
5. Participation coefficient:
The strength of a node is a fundamental measure of prominent importance that can characterize the centrality of a node. Nodes with high strength are often considered as main hubs of the network [49]. An alternative to the strength is the betweenness centrality score, that can also reflect the degree of centrality from another perspective. A high betweenness centrality score for a node indicates that most of the information that flows within a network passes through that node. The local clustering coefficient is another key measure of functional segregation of a node [51]. It quantifies the interconnection of node's neighbours or the possibility that nearby neighbours of a node have also links to each other. The local efficiency reflects the ability of a sub-network constructed by a node and its immediate neighbours to transfer information in terms of the shortest path lengths. For example, a high local efficiency score indicates that the information transmission is more efficient/faster since the shortest paths between them are small. The participation coefficient measures a node's distribution of links/edges among different modules/communities of the graph. When a node doesn't share any link outside its module, then the participation coefficient is zero. On the other hand, if the edges of a node distribute evenly between all modules of a graph, then the coefficient reaches its maximal value. Finally, one can characterize a node based on its participation coefficient as a “provincial” hub, if the score is low, or as a “connector” hub if the score is high [52].
The graph measures were computed utilizing the “igraph” [53] and “brainGraph” packages in the R free software environment [48].
Here, we used two different methodologies/strategies, namely the Least Absolute Shrinkage and Selection Operator (LASSO) and Random Forests (RF) for feature selection. Our final feature matrices were of high dimensionality (the final feature vector size was 420-dimensional: 5 measures for each of the 84 nodes matched to the different anatomical regions of the brain) containing a lot of redundant information. Thus, the choice of the most predominant features is crucial for the classification process and the detection of potential biomarkers of the disease.
The LASSO algorithm has been utilized in recent studies on schizophrenia for both accurate classification and detection of biomarkers of the disease [15], [54].
The procedure of the LASSO algorithm stems by finding a solution to the following optimization problem:
where X represents the feature matrix of size n×p with n being the number of subjects and p the dimensionality of the feature vector, y is a vector containing the class labels for the subjects (0 for healthy controls, 1 for schizophrenia patients), a is the vector of coefficients and λ1 the penalizing factor. The penalty factor λ1 determines how regularized the model is, and thus, how many features are retained. As the value of the penalizing factor drops, coefficients are getting smaller and smaller. Typically, most of them decline to zero. The absolute value of a non zero coefficient can be then used as a measure of the feature's relative importance. It is highly recommended to use LASSO in conjunction with cross-validation [26], [55], [56] (here we used the LOOCV scheme). Thus, one can choose the penalizing factor based on the misclassification error estimated by a CV procedure, so that it is more probable that the final model generalizes well to unknown data samples. Here, we estimated the CV classification error and chose the penalizing factor according to the “one standard deviation rule”. According to this rule [24], we selected the most parsimonious model with its error being no more than one standard deviation above the error of the best model. This practice has been suggested by Hastie et al. [57] for general cross-validation use. For the implementation of LASSO, in conjunction with cross validation, we utilized the “glmnet” package [56] of the R free software environment [48].
RF is an ensemble machine learning algorithm used for both classification and regression purposes [58]. It comprises of a large number of classification and regression trees [59], where each one operates independently. Every individual tree is constructed using a bootstrap (i.e. random sampling with replacement) version of the training data. Once the tree is constructed, the “out of bag” sample (the instances not used in the construction step) of the original data is used as a test set. The error rate in the “out of bag” samples of all trees in the forest is the estimate of the generalization error of the final model. Prediction of new samples are made through a majority voting system between trees where the class with the most votes becomes the final prediction of the model. While the algorithm is inherently stochastic is considered to be robust to noise and resistant to both overfitting and the presence of outliers [60]. The algorithm has been applied to fMRI data for both feature selection and classification purposes [61], [62]. RF, can be also utilized for feature selection using random subspace methodology for measuring feature importance by calculating the so-called Gini impurity index [63]. This index/criterion is computed based on the impurity reduction principle [64] and make no hypothesis of data belonging to specific distributions and therefore,is non parametric. For a binary split, the Gini index of a node t can be calculated as follows:
where p is the frequency of each class j that passes through that node. A low Gini index implies that the specific feature is important in partitioning data into the two distinct classes (here, schizophrenia patient/healthy control). Specifically, a tree structure 𝒯 trained on a learning sample of size N tries to identify at each node t, a split st for which the sample Nt that pass through the node is split into two child nodes tR and tL by maximizing the decrease below [65]:
where
where NT is the number of trees in the forest,
MDG reflects the average of a variable's total decrease in node impurity, weighted by the proportion of samples reaching that node across all trees of the ensemble. In order to have a robust evaluation of feature importance it is generally recommended that the RF should be run multiple times [66]. After measuring and ranking features by importance, elimination of bottom less useful features may lead to an increase in the accuracy of the final model [61]. In this study, we perform feature selection via RF in two steps:
For our computations, we used the R package “randomForest” [67]. The number of trees in the “forest” was set to 500 (similar number of trees also used in [61], [62]) and the parameter concerning the number of features analyzed at each node to find the best split was set equal to the square root of the number of features. The latter is the recommended value of the package and the same strategy have been also used by other studies [61], [62], [66].
The overall pipeline proposed in this study (1A) along with the classification scheme (1B) used is presented in Figure 1. At first, we pre-processed the raw fMRI data (see 2.2) and extracted the time series using an anatomical parcellation scheme (the Desikan-Killiany atlas) for each subject (see 2.2). As a next step, we constructed the cross correlation matrices of the derived time series (see 2.3). We then embedded the dimension of the matrices using ISOMAP and constructed the graph objects of the low dimensional embeddings (this step is skipped in the case of the correlation method). We then applied proportional thresholding (PT) on the graph structures and computed the 5 key local graph-theoretic measures (see 2.5) as described in the methodology. To ensure that the selected features can be generalized across subjects [20], and that the evaluation of the model's performance will be unbiased [24], we performed a double cross validation procedure [19]. Our double cross validation consisted of an outer and an inner loop of “leave one out” cross validation (LOOCV) scheme. The outer loop evaluates the model's performance, while the inner loop optimizes the feature selection procedure (here with LASSO and RF). Initially, out of the total number of subjects considered in this study (N = 145), we first left one subject out (as test subject) and continued with the other 144 (for training and validation). On these remaining subjects (N = 144) we employed the feature selection with another (inner) loop of LOOCV. We then trained a linear standard SVM with the features determined by the inner loop of LOOCV and tried to predict the class label of the initially left/unseen test subjects. This procedure is repeated 145 times. Thus, the estimation of the final model's performance remains unbiased and the selected features are more likely to be generalizable for unseen samples [19], [20]. A schematic representation of the procedure discussed above can be inspected in 1B.
The confusion matrix was also computed for the classification model. In the case of binary classification, the confusion matrix is a 2 × 2 square matrix reporting the number of true positives TP, false positives FP, true negatives TN and false negatives FN. In particular, we considered cases of schizophrenia patients as positives P and healthy controls as negatives N. Sensitivity (known also as the True Positive Rate) and specificity (True Negative Rate) are statistical measures for the evaluation of a binary classification model. The sensitivity TPR is defined as
The classification performance was assessed in the outer loop of LOOCV using standard Linear Support Vector Machines (LSVM). The features given to the classifier were those features determined by the optimization of feature selection procedure inside the inner loop of LOOCV. The final “best” LSVM model was trained 145 times on 144 out of 145 subjects and each time we tried to predict the class label of the unseen test subject. Briefly, the LSVM finds the best plane or hyperplane that separates the two groups in the feature space. Specifically, given a set of data points
Following the methodology described in section 2.3, we first constructed the FCN by correlating the time-courses (matched to the 84 anatomical brain regions) and then we proceeded with the construction of FCN based on the ISOMAP.
As described in the methodology, the embedding dimension was selected via the inspection of the eigenspectrum of the decomposition and complementary by inspecting the residual variance for each one of the low dimensional embeddings. In Figure 2A, we show the average differences between the fifteen largest eigenvalues of the final decomposition, while in 2B the average residual variance across subjects. As it is shown in Figure 2A, there is a large gap between the first and second pair of eigenvalues, while a second gap appears between the second and the third eigenvalues. Finally, a smaller third gap appears between the third and fourth pair of eigenvalues. Finally, after the fifth and sixth eigenvalues, most of the pairwise differences have an almost equal value that is close to zero. Similarly, the average residual variance (Figure 2B) falls rapidly until the first 3 dimensions and continues decreasing smoother and smoother up to five dimensions. In fact, this indicates that there is no substantial difference in the magnitude of eigenvalues (and the decrease in the residual variance) further from this point. Thus, we decided not to explore low-dimensional embeddings larger than five dimensions. Instead, we focused our analysis on four low-dimensional embeddings where 2,3,4 and 5 dimensions are retained. In Table 1, we report the residual variance per embedding dimension for each group. In Figure 3, we present a 2D low dimensional embedding of the average correlation matrix for each one of the two groups (healthy controls and schizophrenia patients) as derived by ISOMAP. For visualization purposes, we included only 46 out of the 84 brain regions which belong to four major Resting state networks namely the Default Mode Network (DMN), the Sub-cortical Limbic Network (SLN), the Sensory Motor Network (SRMN) and the Visual Network (VSN). We label as DMN the lateral parts of the isthmus of cingulate gyrus (ic), lateral orbito-frontal cortex (lof), medial orbito-frontal cortex (mof), parahippocampal gyrus (ph), posterior cingulate cortex (pcc), Precuneus cortex (prec), rostral anterior cingulate cortex (rac). SLN includes the lateral parts of the accubens (acc), amygdala (amg), caudate nucleus (caud), pallidum (pal), putamen (put) and thalamus (thal). SMRN comprises of lateral parts of paracentral gyrus (pac), postcentral gyrus (pc), precentral gyrus (prc) and superior parietal (sp). Finally VSN includes lateral parts of the Cuneus (cun), fusiform gyrus (fs), lateral Occipital cortex (loc), lingual gyrus (lg) and pericalcarine cortex (prcn). As it can be seen from Figure 3, ISOMAP achieves a satisfactory grouping of the four major RSNs in the case of controls 3A. On the other hand nodes are more disorganized in the case of patients with schizophrenia 3B.
Group | Emb. dimension | Residual variance (Mean ± SD) |
Healthy controls | p = 1 | 0.61 ± 0.11 |
p = 2 | 0.39 ± 0.12 | |
p = 3 | 0.25 ± 0.1 | |
p = 4 | 0.18 ± 0.08 | |
p = 5 | 0.13 ± 0.06 | |
Schizophrenia patients | p = 1 | 0.63 ± 0.12 |
p = 2 | 0.41 ± 0.12 | |
p = 3 | 0.29 ± 0.11 | |
p = 4 | 0.21 ± 0.08 | |
p = 5 | 0.15 ± 0.07 |
Using LASSO, the best, with respect to the embedding dimension, number of k nearest neighbors in the ISOMAP algorithm and the level of proportional thresholding (PT), classification rates along with the sensitivity and specificity rates for each method are shown in Table 2. Similarly, results using the RF method for feature selection are also shown in Table 3.
Method | Emb.dim | Parameter | PT (%) | Accuracy (%) | Sensitivity (%) | Specificity (%) |
Correlation | - | - | 30 | 73.1 | 77.4 | 68.9 |
ISOMAP | p = 2 | k = 14 | 40 | 75.9 | 83.1 | 68.9 |
p = 3 | k = 15 | 35 | 79.3 | 85.9 | 72.9 | |
p = 4 | k = 12 | 40 | 76.6 | 85.9 | 67.6 | |
p = 5 | k = 18 | 45 | 75.9 | 80.3 | 71.6 |
Method | Emb.dim | Parameter | PT (%) | Accuracy (%) | Sensitivity (%) | Specificity (%) |
Correlation | - | - | 40 | 71 | 77.4 | 64.8 |
ISOMAP | p = 2 | k = 15 | 45 | 76.6 | 80.3 | 72.9 |
p = 3 | k = 15 | 35 | 78.6 | 87.3 | 70.3 | |
p = 4 | k = 12 | 40 | 74.5 | 81.7 | 67.6 | |
p = 5 | k = 17 | 45 | 76.6 | 81.7 | 71.6 |
Using LASSO for feature selection, the best classification rate for ISOMAP was 79.3%, obtained retaining 3 dimensions, k = 15 nearest neighbours and 35% PT. The conventional correlation peaked at 73.1% (at 30% PT). Interestingly, the 6.2% difference in the classification performance translates mainly to an increase in the sensitivity (reflecting the ability of the model to correctly identify a schizophrenic subject). Using RF for feature selection the results were similar to those obtained with LASSO. Specifically, the correlation method peaked at 73% (at 40% PT) while ISOMAP at 78.6% and 35% PT. The whole pattern of classification rates, using both strategies of feature selection, through all PT points used in this study is shown in Figure 4A,B. Using both feature selection methodologies, ISOMAP did not only produced the best classification rates but was also more robust with respect to the level of thresholding when compared to the standard methodology. When using LASSO 4A, ISOMAP was more robust than the correlation method for a wide range of levels of PT (eg. 30%–50%) with the accuracy rates being above 75%. When using RF for feature selection, ISOMAP provided again superior results with accuracy rates being higher than 70% in the PT range of 30% to 50%. Figure 5 depicts the maximum classification obtained for each low dimensional embedding (2, 3, 4 and 5 dimensions retained) with respect to the value of k nearest neighbors for both of the feature selection strategies employed in this study (eg LASSO and RF). All four ISOMAP-based low-dimensional embeddings produced better classification rates than the standard cross-correlation, while the overall optimal was obtained when using three embedding dimensions for both feature selection methods.
Despite the fact that the final feature vector for each subject was 420-dimensional (five local measures for each one of the 84 brain regions) with the total number of subjects being 145, the LASSO selected mainly, two features. The selected features which were chosen almost equally for both ISOMAP and correlation were the participation coefficient of the right thalamus and the strength of the right lingual gyrus. In
In general, when using the Random Forest (RF) algorithm for feature selection, more features were selected comparing to LASSO. Barplots of selected features when using the RF algorithm are presented in
Finally, for the two most important features (the ones derived by ISOMAP and led to the highest accuracy rate ), we present the distributions between groups as boxplots (Figure 8A,B). Specifically, it is shown that controls exhibited a larger strength in the right lingual gyrus (Welch's t-test: p < 0.05 Bonferroni corrected for multiple comparisons over 420 features) while patients exhibited larger participation coefficients of the right thalamus (Welch's t-test: p < 0.05 Bonferroni corrected for multiple comparisons over 420 features). We also show a projection of each subject on a 2D feature space as derived by these 2 features (Figure 8C). Despite the fact that it is clear that these two features provide a good classification between groups, the cluster of patients is more tight than the cluster of healthy controls. This observation indicates that our model tends to provide a relatively high sensitivity rate and a more modest specificity rate.
Our analysis targeted at revealing classification biomarkers for schizophrenia patients at the ROI level based on resting state fMRI recordings using ISOMAP for the construction of embedding FCN. For our analysis, we used the publicly available dataset (COBRE) matching the brain anatomies to the Desikan-Killiany brain atlas. To assess the classification performance of the FCN constructed with ISOMAP, we compared it against the standard cross-correlation technique on a double cross-validation basis. When using the standard cross-correlation approach, we got similar results with the ones reported in previous studies [18], [19], [69], [70]. For example, Moghimi et al. [19] used correlation to analyse a similarly large independent rsfMRI dataset of 170 subjects (88 healthy controls and 82 schizophrenia patients) using double cross-validation for both feature selection and model validation, getting classification rates as high as 73%. In the study of [19] that employed the correlation method for constructing FCN from rsfMRI matching to anatomical regions of the brain (as in our work), the highest classification rate obtained using a single feature. In particular, this feature was the matching index between the left thalamus and the left post central gyrus; the thalamus is part of the reward system while the post central gyrus is located in the primary somatosensory cortex, which is part of the somatosensory system. The best accuracy rate was 73% and in the same threshold point as the one we found here (preserving 30% of the strongest edges). Moreover, the sensitivity and specificity rates reported in [19] were close enough with the ones reported here when using the cross-correlation method and LASSO for feature selection. In particular, they reported a sensitivity rate of 77% (here, 77.4%) and specificity rate of 68% (here 68.9%) using SVM. This is remarkable, as we worked with an independent dataset and tested different set of features utilizing different feature selection methods. In a recent study [21], the authors applied a classification framework proposed in [22] to two independent datasets evaluating the within and between site generalizability of the model. While the proposed methodology in [22] reported a satisfying 93% accuracy rate, Cai et al. [21] found a within-site accuracy rate of 73% and between-site accuracy of 70%. The approximately 20 % difference was also found in the study of [19] that is attributed to the differences of the results taken when using single and double cross validation. Single cross validation tended to over-optimistically evaluate the model's performance [19], [20]. Using the standard cross-correlation and double cross validation we report practically the same classification accuracy with the one reported in [19] working on an independent dataset. In another study of Nieuwenhuis et al. [69], two large independent datasets were analysed. Using T1 structural MR images and double cross-validation, they reported a 70.4% classification accuracy. Another more recent study [71] involving also two large independent fMRI datasets tested different strategies of Group Independent component analysis for the derivation of subject specific networks from group networks. They used these networks as features and the best reported average classification accuracy was 76.5% (evaluating the model with 100 repeats of cross validation). These classification rates are more likely to reflect the true classification power that contemporary models have [20], [21]. [23]–[25].
Our feature selection analysis based on both the LASSO and Random Forest algorithms revealed that out of a 420-dimensional feature vector for each subject, only a handful of features were important. Thus, there was a great amount of redundancy inherent to the dataset, a fact that was also reported in [19] (where just one single feature produced the best accuracy rates), in [70] (where only seven discriminative independent components used as features) and in [18] (where ten features were found as important, namely the betweenness centrality scores of ten prominent hubs of the network).
On the other hand, ISOMAP has been recently applied for the construction of FCN [16], [27] with the nodes of the network matching the outcomes of a single-subject ICA analysis. [16], working on the same dataset as here, reported a 65% classification accuracy using SVM, considering 13 global graph theoretic measures (no feature selection was performed, thus all these measures were given as features to the classifier). The model's performance was evaluated using a 10-fold cross validation scheme. Here, building on this work and on a recently published work (see [27]) which targeted at the global properties of the FCN using ICA, we made a more thorough assessment of ISOMAP, focusing on the analysis of the local properties of the embedded FCN, thus being able to identify classification biomarkers linked with the activity in specific brain regions. First, we used an anatomical atlas to associate the rsfMRI time series with specific brain regions in order to address the well known problem of the variability in the ICA decomposition when used for the construction of FCN [27], [72]. The experiment comprised of only a single session per subject with a relatively small duration (6 minutes), so we wouldn't expect a robust decomposition for all subjects (see also the discussion in [73]). Second, for our analysis we used key local graph theoretic-measures (strength of node, local clustering, local efficiency, participation coefficient and betweenness centrality) and performed feature selection using LASSO and Random Forests. Third, we considered a wide range of values for tuning the parameter the number k of nearest neighbours in ISOMAP and justified the choice of the low-dimensional embedding dimension based on the gap in the eigenspectrum and the residual variance. Fourth, we took an “extra” advanced pre-processing step by using ICA AROMA [37] to detect and factor out noise-related (motion artifacts and other structured noise components like cardiac pulsation confounds) independent components as it is highly recommended (see the discussion in [16]). Fifth, we compared ISOMAP to the current standard cross-correlation (by correlating the time series and performed thresholding to construct FCN), based at the local theoretical graph measures of the derived FCN.
Our analysis showed that ISOMAP outperformed the standard cross-correlation approach, thus increasing the classification performance by 6.2%. This difference was translated mainly to an increase in the sensitivity rate (the ability of a classification model to correctly identify a schizophrenic subject). The sensitivity rate for ISOMAP was 85.9% compared to a 77.4% for the conventional method. Furthermore, the performance of ISOMAP was more robust producing higher classification rates than the conventional methodology across different threshold points. As ISOMAP needs to be tuned (k-nearest neighbours, embedding dimension p), we showed that for a considerable range of the values of k, ISOMAP scored higher classification rates (above 75%). All low-dimensional embeddings produced higher classification rates than the competent standard approach. Finally, when using ISOMAP, both of the feature selection methods had a tendency to choose a more simple model (with mainly two or three features, appearing in almost all 145 computational experiments). This indicates that ISOMAP captured most of the useful information in the dataset (by reducing it to its intrinsic dimension), while discarded information that could be attributed to various sources of noise and confounds (see also the discussion in [46]). At this point, we should note that we decided to use a simple “baseline” classifier (such as the LSVM) as the aim of this study was the investigation of the performance of ISOMAP compared to the standard cross-correlation and not the influence of various machine learning approaches. Consequently, our analysis was focused on how the ISOMAP-based derived embedded FCN affect the feature selection procedure as implemented here by Lasso and Random Forests. Finally, we used a double cross-validation scheme to get an unbiased estimate of the expected accuracy of the model (i.e. not to get a specific classification model [23]) optimizing the feature selection procedure. A comparative analysis of different machine learning algorithms is out of the scope of the current study (such a comparative analysis between various manifold and machine learning approaches, yet based on global-graph theoretical properties of ICA-based constructed FCN can be found in [27]).
Using ISOMAP for the construction of brain atlas-based embedded FCN and utilizing different feature selection methods, we found that the most informative features that led to the highest classification rates were the participation coefficient of the right thalamus and the strength of the right lingual gyrus. On the one hand, the thalamus is thought to play a prominent role in the coordination of information as it flows from functional and structural pathways which have been consistently linked to the schizophrenia [74], [75]. Abnormalities in the function of the thalamus have been associated with cognitive deficits and anomalies in sensory experience (e.g. auditory/visual hallucinations) [76]. On the other hand, The lingual gyrus is a main component of the visual cortex that contributes in visual processing/memory [77] and the identification and recognition of words [78]. Many studies have reported abnormalities in the lingual gyrus associated with schizophrenia [79], [80]. Interestingly, recent classification studies involving two independent datasets refer to both of these brain regions as key regions in both characterization of the disease and discrimination between healthy controls and patients with schizophrenia [21], [81]. Other studies have listed these regions as discriminatory in schizophrenia as well [15], [19]. Other important regions found here, include the cuneus and latteral occipital cortex, both parts of the occipital lobe which has been known to be affected as a consequence of the disease [82]. Moreover, our study provides evidence that the use of ISOMAP for the construction of embedded FCN contributes to a more accurate and robust classification plus a finer detection of biomarkers at the ROI level that are more likely to be generalized on other datasets.
[1] |
Roskoski R Jr (2012) ERK1/2 MAP kinases: Structure, function, and regulation. Pharmacol Res 66: 105-143. doi: 10.1016/j.phrs.2012.04.005
![]() |
[2] |
Krishna M, Narang H (2008) The complexity of mitogen-activated protein kinases (MAPKs) made simple. Cell Mol Life Sci 65: 3525-3544. doi: 10.1007/s00018-008-8170-7
![]() |
[3] |
Yao Z, Seger R (2009) The ERK signaling cascade–views from different subcellular compartments. Biofactors 35: 407-416. doi: 10.1002/biof.52
![]() |
[4] | Duan RD, Williams JA (1994) Cholecystokinin rapidly activates mitogen-activated protein kinase in rat pancreatic acini. Am J Physiol 267: 401-408. |
[5] | Dabrowski A, Groblewski GE, Schafer C, et al. (1997) Cholecystokinin and EGF activate a MAPK cascade by different mechanisms in rat pancreatic acinar cells. Am J Physiol 273: C1472-C1479. |
[6] |
Daulhac L, Kowalski-Chauvel A, Pradayrol L, et al. (1997) Ca2+ and protein kinase C-dependent mechanisms involved in gastrin-induced Shc/Grb2 complex formation and P44-mitogen-activated protein kinase activation. Biochem J 325: 383-389. doi: 10.1042/bj3250383
![]() |
[7] | Piiper A, Gebhardt R, Kronenberger B, et al. (2000) Pertussis Toxin Inhibits Cholecytoskinin- and Epidermal Growth Factor-Induced Mitogen-Activated Protein Kinase Activation by Disinhibitation of the cAMP Signaling Pathway and Inhibition of c-Raf-1. Mol Pharmacol 58: 608-613. |
[8] |
Piiper A, Elez R, You S, et al. (2003) Cholecystokinin Stimulates Extracellular Signal-regulated Kinase through Activation of the Epidermal Growth Factor Receptor, Yes, and Protein Kinase C. J Biol Chem 278: 7065-7072. doi: 10.1074/jbc.M211234200
![]() |
[9] | Koh Y, Tamizhselvi R, Bhatia M (2009) Extracellular Signal-Regulated Kinase 1/2 and c-Jun NH2-Terminal Kinase, through Nuclear Factor-kB and Activator Protein-1, Contribute to Caerulein-Induced Expression of Substance P and Neurokinin-1 Receptors in Pancreatic Acinar Cells. JPET 332: 940-948. |
[10] |
Williams JA (2008) Receptor-mediated signal transduction pathways and the regulation of pancreatic acinar cell function. Curr Opin Gastroenterol 24: 573-579. doi: 10.1097/MOG.0b013e32830b110c
![]() |
[11] | Jacob C, Bunnet NW (2006) Transmembrane Signaling by G Protein-Coupled Receptors, In: Physiology of the Gastrointestinal Tract, 4 Eds., Academic Press, 63-85. |
[12] | Williams JA, Yule DI (2012) Stimulus-secretion Coupling in Pancreatic Acinar Cells, In: Physiology of the Gastrointestinal Tract, 5 Eds., Elsevier, 1361-1398. |
[13] |
Ramos JW (2008) The regulation of extracellular signal-regulated kinase (ERK) in mammalian cells. Int J Biochem Cell Biol 40: 2707-2719. doi: 10.1016/j.biocel.2008.04.009
![]() |
[14] | Nicke B, Tseng MJ, Fenrich M, et al. (1999) Adenovirus-mediated gene transfer of RasN17 inhibits specific CCK actions on pancreatic acinar cells. Am Physiol 276: 499-506. |
[15] |
Nishizuka Y (1992) Intracellular signaling by hydrolysis of phospholipids and activation of protein kinase C. Science 258: 607-614. doi: 10.1126/science.1411571
![]() |
[16] | Newton AC (2009) Protein kinase C: poised to signal. Am J Physiol Endocrinol Metab 298: E395-E402. |
[17] |
Mochly-Rosen D (1995) Localization of protein kinases by anchoring proteins: a theme in signal transduction. Science 268: 247-251. doi: 10.1126/science.7716516
![]() |
[18] | Mochly-Rosen D, Wu G, Hahn H, et al. (2000) Cardiotrophic effects of protein kinase C-analysis in vivo modulation of PKCε Translocation. Circ Res 86: 1172-1179. |
[19] |
Wu-Zhang AX, Newton AC (2013) Protein kinase C pharmacology: refining the toolbox. Biochem J 452: 195-209. doi: 10.1042/BJ20130220
![]() |
[20] |
Churchill EN, Qvit N, Mochly-Rosen D (2009) Rationally designed peptide regulators of protein kinase C. Trends Endocrinol Metab 20: 25-33. doi: 10.1016/j.tem.2008.10.002
![]() |
[21] |
Bastani B, Yang L, Baldassare JJ, et al. (1995) Cellular distribution of isoforms of protein kinase C (PCK) in pancreatic acini. Biochimica et Biophysica Acta 1269: 307-315. doi: 10.1016/0167-4889(95)00120-0
![]() |
[22] |
Kim MJ, Lee YS, Lee KH, et al. (2001) Site-specific localization of protein kinase C isoforms in rat pancreas. Pancreatology 1: 36-42 doi: 10.1159/000055790
![]() |
[23] |
Li C, Chen X, Williams JA (2004) Regulation of CCK-induced amylase release by PKC-δ in rat pancreatic acinar cells. Am J Physiol Gastrointest Liver Physiol 287: G764-G771. doi: 10.1152/ajpgi.00111.2004
![]() |
[24] |
Rodriguez-Martin E, Boyano-Adanex MC, Bodega G, et al. (1999) Redistribution of protein kinase C isoforms in rat pancreatic acini during lactation and weaning. FEBS Lett 445: 356-360. doi: 10.1016/S0014-5793(99)00133-7
![]() |
[25] |
Fleming AK, Storz P (2017) Protein kinase C isoforms in the normal pancreas and in pancreatic disease. Cell Signal 40: 1-9 doi: 10.1016/j.cellsig.2017.08.005
![]() |
[26] |
Braz JC, Bueno OF, De Windt LJ, et al. (2002) PKC alpha regulates the hypertrophic growth of cardiomyocytes through extracellular signal-regulated kinase1/2 (ERK1/2). J Cell Biol 156: 905-919. doi: 10.1083/jcb.200108062
![]() |
[27] |
Chen X, Edwards JA, Logsdon CD, et al. (2002) Dominant negative Rab3D inhibits amylase release from mous pancreatic acini. J Biol Chem 277: 18002-18009. doi: 10.1074/jbc.M201248200
![]() |
[28] |
Besson A, Davy A, Robbins SM, et al. (2001) Differential activation of ERKs to focal adhesions by PKC epsilon is required for PMA-induced adhesion and migration of human glioma cells. Oncogene 20: 7398-7407. doi: 10.1038/sj.onc.1204899
![]() |
[29] |
Ginnan R, Pfleiderer PJ, Pumiglia K, et al. (2004) PKC-delta and CaMKII-delta 2 mediate ATP-dependent activation of ERK1/2 in vascular smooth muscle. Am J Physiol Cell Physiol 286: C1281-C1289. doi: 10.1152/ajpcell.00202.2003
![]() |
[30] |
Kampfer S, Windegger M, Hochholdinger F, et al. (2001) Protein kinase C isoforms involved in the transcriptional activation of cyclin D1 by transforming Ha-Ras. J Biol Chem 276: 42834-42842. doi: 10.1074/jbc.M102047200
![]() |
[31] | Torricelli C, Valacchi G, Maioli E (2001) Novel PKCs activate ERK through PKD1 in MCF-7 cells. In Vitro Cell Dev Bio-Animal 47: 73-81. |
[32] |
Olson ER, Shamhart PE, Naugle JE, et al. (2008) Angiotensin II-induced extracellular signal-regulated kinase 1/2 activation is mediated by protein kinase Cdelta and intracellular calcium in adult rat cardiac fibroblasts. Hypertension 51: 704-711. doi: 10.1161/HYPERTENSIONAHA.107.098459
![]() |
[33] |
Gschwendt M, Muller HJ, Kielbassa K, et al. (1994) Rottlerin, a novel protein kinase inhibitor. Biochem Biophys Res Commun 199: 93-98. doi: 10.1006/bbrc.1994.1199
![]() |
[34] | Martiny-Baron G, Kazanietz MG, Mischak H, et al. (1993) Selective inhibition of protein kinase C isozymes by the indolocarbazol Go 6976. J Biol Chem 268: 9194-9197. |
[35] |
Tapia JA, Jensen RT, Garcia-Marin LJ (2006) Rottlerin inhibits stimulated enzymatic secretion and several intracellular signaling transduction pathways in pancreatic acinar cells by a non-PKC-delta-dependent mechanism. Biochim Biophys Acta 1763: 25-38. doi: 10.1016/j.bbamcr.2005.10.007
![]() |
[36] |
Chen L, Hahn H, Wu G, et al. (2001) Opposing cardioprotective actions and parallel hypertrophic effects of δPKC and εPKC. PNAS 98: 11114-11119. doi: 10.1073/pnas.191369098
![]() |
[37] |
Churchill E, Budas G, Vallentin A, et al. (2008) PKC isozymes in chronic cardiac disease: possible therapeutic targets. Annu Rev Pharmacol Toxicol 48: 569-599. doi: 10.1146/annurev.pharmtox.48.121806.154902
![]() |
[38] |
Chen L, Mochly-Rosen D (2001) Opposing effects δ of ξ and PKC in ethanol-induced cardioprotection. J Mol Cell Cardiol 33: 581-585. doi: 10.1006/jmcc.2000.1330
![]() |
[39] |
Budas GR, Churchill EN, Mochly-Rosen D (2007) Cardioprotective mechanisms of PKC isozyme-selective activators and inhibitors in the treatment of ischemia-reperfusion injury. Pharm Res 55: 523-536. doi: 10.1016/j.phrs.2007.04.005
![]() |
[40] |
Sabbatini ME, Chen X, Ernst SA, et al. (2008) Rap1 activation plays a regulatory role in pancreatic amylase secretion. J Biol Chem 283: 23884-23894. doi: 10.1074/jbc.M800754200
![]() |
[41] |
Corbit KC, Trakul N, Eves EM, et al. (2003) Activation of Raf-1 signaling by protein kinase C through a mechanism involving Raf Kinase Inhibitory Protein. J Biol Chem 278: 13061-13068. doi: 10.1074/jbc.M210015200
![]() |
[42] | Han B, Ji B, Logsdon CD (2001) CCK independently activates intracellular trypsinogen and NF-kappaB in rat pancreatic acinar cells. Am J Physiol Cell Physiol 180: C465-C472. |
[43] | Satoh A, Gukovskaya AS, Nieto JM, et al. (2004) PKC-delta and -epsilon regulate NF-kappaB activation induced by cholecystokinin and TNF-alpha in pancreatic acinar cells. Am J Physiol Gastrointest Liver Physiol 387: G582-G591. |
[44] |
Thrower EC, Osgood S, Shugrue CA, et al. (2008) The novel protein kinase C isoforms -delta and -epsilon modulate caerulein-induced zymogen activation in pancreatic acinar cells. Am J Physicol Gastrointest Liver Physiol 294: G1344-1353. doi: 10.1152/ajpgi.00020.2008
![]() |
[45] |
Uchida T, Iwashita N, Ohara-Imaizumi M, et al. (2007) Protein kinase Cδ plays a non-redundant role in insulin secretion in pancreatic beta cells. J Biol Chem 282: 2707-2716. doi: 10.1074/jbc.M610482200
![]() |
[46] |
Thrower EC, Wang J, Cheriyan S, et al. (2009) Protein kinase C δ-mediated processes in cholecystokinin-8-stimulated pancreatic acini. Pancreas 38: 930-935. doi: 10.1097/MPA.0b013e3181b8476a
![]() |
[47] |
Cosen-Binker LI, Lam PP, Binker MG, et al. (2007) Alcohol/cholecystokinin-evoked pancreatic acinar basolateral exocytosis is mediated by protein kinase C alpha phosphorylation of Munc18c. J Biol Chem 282: 13047-13058. doi: 10.1074/jbc.M611132200
![]() |
1. | I.K. Gallos, L. Mantonakis, E. Spilioti, E. Kattoulas, E. Savvidou, E. Anyfandi, E. Karavasilis, N. Kelekis, N. Smyrnis, C.I. Siettos, The relation of integrated psychological therapy to resting state functional brain connectivity networks in patients with schizophrenia, 2021, 306, 01651781, 114270, 10.1016/j.psychres.2021.114270 | |
2. | Jacob Levman, Maxwell Jennings, Ethan Rouse, Derek Berger, Priya Kabaria, Masahito Nangaku, Iker Gondra, Emi Takahashi, A morphological study of schizophrenia with magnetic resonance imaging, advanced analytics, and machine learning, 2022, 16, 1662-453X, 10.3389/fnins.2022.926426 | |
3. | Pan Zhang, Zhaoxuan He, Yangke Mao, Ruirui Sun, Yuzhu Qu, Li Chen, Peihong Ma, Shuai Yin, Tao Yin, Fang Zeng, Aberrant resting-state functional connectivity and topological properties of the subcortical network in functional dyspepsia patients, 2022, 15, 1662-5099, 10.3389/fnmol.2022.1001557 | |
4. | Evangelos Galaris, Gianluca Fabiani, Ioannis Gallos, Ioannis Kevrekidis, Constantinos Siettos, Numerical Bifurcation Analysis of PDEs From Lattice Boltzmann Model Simulations: a Parsimonious Machine Learning Approach, 2022, 92, 0885-7474, 10.1007/s10915-022-01883-y | |
5. | Małgorzata Plechawska-Wójcik, Paweł Karczmarek, Paweł Krukow, Monika Kaczorowska, Mikhail Tokovarov, Kamil Jonak, Recognition of Electroencephalography-Related Features of Neuronal Network Organization in Patients With Schizophrenia Using the Generalized Choquet Integrals, 2021, 15, 1662-5196, 10.3389/fninf.2021.744355 | |
6. | Nikita Pospelov, Alina Tetereva, Olga Martynova, Konstantin Anokhin, The Laplacian eigenmaps dimensionality reduction of fMRI data for discovering stimulus-induced changes in the resting-state brain activity, 2021, 1, 26669560, 100035, 10.1016/j.ynirp.2021.100035 | |
7. | Zhiyi Chen, Xuerong Liu, Qingwu Yang, Yan-Jiang Wang, Kuan Miao, Zheng Gong, Yang Yu, Artemiy Leonov, Chunlei Liu, Zhengzhi Feng, Hu Chuan-Peng, Evaluation of Risk of Bias in Neuroimaging-Based Artificial Intelligence Models for Psychiatric Diagnosis, 2023, 6, 2574-3805, e231671, 10.1001/jamanetworkopen.2023.1671 | |
8. | Nuo Cheng, Meihao Guo, Fang Yan, Zhengjun Guo, Jun Meng, Kui Ning, Yanping Zhang, Zitian Duan, Yong Han, Changhong Wang, Application of machine learning in predicting aggressive behaviors from hospitalized patients with schizophrenia, 2023, 14, 1664-0640, 10.3389/fpsyt.2023.1016586 | |
9. | Zhiyi Chen, Bowen Hu, Xuerong Liu, Benjamin Becker, Simon B. Eickhoff, Kuan Miao, Xingmei Gu, Yancheng Tang, Xin Dai, Chao Li, Artemiy Leonov, Zhibing Xiao, Zhengzhi Feng, Ji Chen, Hu Chuan-Peng, Sampling inequalities affect generalization of neuroimaging-based diagnostic classifiers in psychiatry, 2023, 21, 1741-7015, 10.1186/s12916-023-02941-4 | |
10. | Chang Wang, Yaning Ren, Rui Zhang, Chen Wang, Xiangying Ran, Jiefen Shen, Zongya Zhao, Wei Tao, Yongfeng Yang, Wenjie Ren, Yi Yu, Schizophrenia classification and abnormalities reveal of brain region functional connection by deep-learning multiple sparsely connected network, 2024, 96, 17468094, 106580, 10.1016/j.bspc.2024.106580 | |
11. | Javier Gonzalez-Castillo, Isabel S. Fernandez, Ka Chun Lam, Daniel A. Handwerker, Francisco Pereira, Peter A. Bandettini, Manifold learning for fMRI time-varying functional connectivity, 2023, 17, 1662-5161, 10.3389/fnhum.2023.1134012 | |
12. | Ioannis Gallos, Dimitrios Tryfonopoulos, Gidi Shani, Angelos Amditis, Hossam Haick, Dimitra Dionysiou, Advancing Colorectal Cancer Diagnosis with AI-Powered Breathomics: Navigating Challenges and Future Directions, 2023, 13, 2075-4418, 3673, 10.3390/diagnostics13243673 | |
13. | Ioannis K. Gallos, Daniel Lehmberg, Felix Dietrich, Constantinos Siettos, Data-driven modelling of brain activity using neural networks, diffusion maps, and the Koopman operator, 2024, 34, 1054-1500, 10.1063/5.0157881 | |
14. | Yin Xia Bai, Jia Xin Luo, Duo Peng, Jing Jing Sun, Yi Fang Gao, Li Xia Hao, B. G. Tong, Xue Mei He, Jia Yu Luo, Zi Hong Liang, Fan Yang, Brain network functional connectivity changes in long illness duration chronic schizophrenia, 2024, 15, 1664-0640, 10.3389/fpsyt.2024.1423008 | |
15. | Shiyue Li, Yating Xiao, Weichen Yuan, 2024, Chapter 6, 978-981-97-4389-6, 67, 10.1007/978-981-97-4390-2_6 | |
16. | Tushar Hrishikesh Jaware, Chittaranjan Nayak, Priyadarsan Parida, Nawaf Ali, Yogesh Sharma, Wael Hadi, Enhanced Neonatal Brain Tissue Analysis via Minimum Spanning Tree Segmentation and the Brier Score Coupled Classifier, 2024, 13, 2073-431X, 260, 10.3390/computers13100260 | |
17. | Tahmineh Azizi, Time varying analysis of dynamic resting-state functional brain network to unfold memory function, 2024, 4, 27725286, 100148, 10.1016/j.neuri.2023.100148 | |
18. | Mahdi Mohammadkhanloo, Mohammad Pooyan, Hamid Sharini, 2024, Identification of Schizophrenia using Functional Connectivity and Graph Theory through Resting State Functional Magnetic Resonance Imaging (rs- fMRI), 979-8-3503-4887-3, 194, 10.1109/QICAR61538.2024.10496660 | |
19. | Fabiana Santana Kragelund, Konstantinos Spiliotis, Marco Heerdegen, Tina Sellmann, Henning Bathel, Anika Lüttig, Angelika Richter, Jens Starke, Rüdiger Köhling, Denise Franz, [Network-wide effects of pallidal deep brain stimulation normalised abnormal cerebellar cortical activity in the dystonic animal model, 2025, 205, 09699961, 106779, 10.1016/j.nbd.2024.106779 |
Group | Emb. dimension | Residual variance (Mean ± SD) |
Healthy controls | p = 1 | 0.61 ± 0.11 |
p = 2 | 0.39 ± 0.12 | |
p = 3 | 0.25 ± 0.1 | |
p = 4 | 0.18 ± 0.08 | |
p = 5 | 0.13 ± 0.06 | |
Schizophrenia patients | p = 1 | 0.63 ± 0.12 |
p = 2 | 0.41 ± 0.12 | |
p = 3 | 0.29 ± 0.11 | |
p = 4 | 0.21 ± 0.08 | |
p = 5 | 0.15 ± 0.07 |
Method | Emb.dim | Parameter | PT (%) | Accuracy (%) | Sensitivity (%) | Specificity (%) |
Correlation | - | - | 30 | 73.1 | 77.4 | 68.9 |
ISOMAP | p = 2 | k = 14 | 40 | 75.9 | 83.1 | 68.9 |
p = 3 | k = 15 | 35 | 79.3 | 85.9 | 72.9 | |
p = 4 | k = 12 | 40 | 76.6 | 85.9 | 67.6 | |
p = 5 | k = 18 | 45 | 75.9 | 80.3 | 71.6 |
Method | Emb.dim | Parameter | PT (%) | Accuracy (%) | Sensitivity (%) | Specificity (%) |
Correlation | - | - | 40 | 71 | 77.4 | 64.8 |
ISOMAP | p = 2 | k = 15 | 45 | 76.6 | 80.3 | 72.9 |
p = 3 | k = 15 | 35 | 78.6 | 87.3 | 70.3 | |
p = 4 | k = 12 | 40 | 74.5 | 81.7 | 67.6 | |
p = 5 | k = 17 | 45 | 76.6 | 81.7 | 71.6 |
Group | Emb. dimension | Residual variance (Mean ± SD) |
Healthy controls | p = 1 | 0.61 ± 0.11 |
p = 2 | 0.39 ± 0.12 | |
p = 3 | 0.25 ± 0.1 | |
p = 4 | 0.18 ± 0.08 | |
p = 5 | 0.13 ± 0.06 | |
Schizophrenia patients | p = 1 | 0.63 ± 0.12 |
p = 2 | 0.41 ± 0.12 | |
p = 3 | 0.29 ± 0.11 | |
p = 4 | 0.21 ± 0.08 | |
p = 5 | 0.15 ± 0.07 |
Method | Emb.dim | Parameter | PT (%) | Accuracy (%) | Sensitivity (%) | Specificity (%) |
Correlation | - | - | 30 | 73.1 | 77.4 | 68.9 |
ISOMAP | p = 2 | k = 14 | 40 | 75.9 | 83.1 | 68.9 |
p = 3 | k = 15 | 35 | 79.3 | 85.9 | 72.9 | |
p = 4 | k = 12 | 40 | 76.6 | 85.9 | 67.6 | |
p = 5 | k = 18 | 45 | 75.9 | 80.3 | 71.6 |
Method | Emb.dim | Parameter | PT (%) | Accuracy (%) | Sensitivity (%) | Specificity (%) |
Correlation | - | - | 40 | 71 | 77.4 | 64.8 |
ISOMAP | p = 2 | k = 15 | 45 | 76.6 | 80.3 | 72.9 |
p = 3 | k = 15 | 35 | 78.6 | 87.3 | 70.3 | |
p = 4 | k = 12 | 40 | 74.5 | 81.7 | 67.6 | |
p = 5 | k = 17 | 45 | 76.6 | 81.7 | 71.6 |